EXECUTIVE SUMMARY
Climate and weather are the consequences of a complex physical/chemical/biological system linking the atmosphere, land, and oceans.
Physically and dynamically, the system comprises many components and interactions of the energy and water cycles. Chemically and biologically,
the system is governed by the cycling of carbon, sulphur, nitrogen and oxygen. The components of the system are interconnected since radiatively
active greenhouse gases (GHGs) and aerosols (e.g. sulphates and black carbon) directly and indirectly affect the energy and hydrological budgets,
and, hence global climate. Canada and a number of other developed nations have invested in the ongoing development of comprehensive coupled climate
models in order to understand and forecast climate change. At the time of the drafting of the Third Assessment Report of the IPCC none of the models
used to make projections of future climate included carbon as a prognostic variable. In those simulations, GHG concentrations were prescribed and the
resulting climate change does not “feedback” on the rate of ocean and terrestrial C cycling and, hence, on the GHG concentrations themselves. It is
important to include the oceanic and terrestrial biospheric carbon fluxes as prognostic variables in comprehensive coupled climate models to properly
represent these feedbacks. Our objectives are to:
- develop and test an integrated carbon cycle model including land, ocean, and atmospheric components
for inclusion in the CCCma CGCM;
- incorporate and test the coupled C-cycle model in CGCM simulations directed toward transient climate change
calculations with C as a prognostic (forecast) variable;
- perform model studies of the role of carbon dioxide (CO2) and methane (CH4), the two
most important GHGs, in order to investigate how the their cycle might respond to a changing climate through dynamic feedbacks.
Attaining these objectives represents a significant and important scientific challenge. The representation of the terrestrial C cycle will be developed
in parallel with, and closely coupled to, representations of the surface energy and hydrological processes in CLASS, the Canadian Land Surface Scheme
used in the CGCM. It will compliment and build on work done on C-CLASS, an incomplete C version of CLASS developed through the LSP node of the Climate
Research Network (CRN). It will include plant functional types, dynamic carbon allocation, a simple N cycle, vegetation dynamics (i.e. ecosystem
disturbance and land-use change), and trace gases. The ocean C cycle, including inorganic carbon and a simple treatment of the oceanic ecosystem,
will be incorporated into the ocean component of the CGCM, building on preliminary work begun in the Carbon node of the CRN. The atmospheric component
will include CO2 and CH4 concentrations as prognostic variables. The new coupling code, developed to allow more efficient calculation on multi-processor
super-computers, will be expanded to accommodate the addition of C-cycle components.
We have divided the project into two phases, with year 3 and year 5 milestones. During Phase I we will develop and evaluate the first version of the
carbon components and will implement these into the CGCM. Anthropogenic emissions of CO2 and CH4 will be specified, their concentrations will be allowed
to equilibrate over multi-year model integration, and the results will be analyzed to evaluate the coupled model performance. During Phase II, a similar
strategy will be adopted, but expanded versions of the C-cycle components (e.g. introduction of landscape dynamics to the terrestrial model) will be
implemented in the CGCM. Phase II will culminate in a climate change integration with fully interactive C components.
The policy relevance of this work is immediate. If an active C-cycle is found to reduce the ability of the terrestrial biosphere and/or the oceans to
take up future excess GHGs, then proposed policies of greenhouse gas management that rely on the ‘natural’ cycling of C will have to be rethought.
Even if ocean and terrestrial systems are persistent sinks, the concentration of GHGs, even in the ‘best case’ emission scenario, will stabilize at
double or triple the present concentrations. The proposed Canadian Coupled Carbon Climate Model (GC3M) model will be capable of simulating the climate
implications of different emission scenarios, and will, therefore, assist in forming policies related to mitigation and adaptation, and help identify
vulnerabilities.
Note to readers of this proposal:
The proposed research program has been written for five years based on the time we feel it will take to develop
a fully coupled carbon – climate model. However, we have clearly identified three and five year objectives. The three-year objectives and science plan
that we are initially seeking funding for can, therefore, be viewed in the context of the overall project. If we are funded for three years and we are
successful in attaining most of our year 3 milestones we expect to apply for two additional years of support from CFCAS.
go to top
PLANNING
This research network has several roots. The first and most obvious is the strength that Canada has at the Canadian Centre for Climate Modelling and
Analysis (CCCma) in climate modelling. The CCCma research team has played a major role in the scientific assessments of climate change through the
auspices of the Intergovernmental Panel on Climate Change (IPCC). The Climate Research Network (CRN) was organized to help coordinate university and
government based climate scientists to further develop and enhance specific components of the CCCma Coupled General Circulation Model (CGCM). As new
knowledge arises and as new policy initiatives demand scientific input and assessment, further developments in the GCM are required. The CRN has supported
two initiatives to enhance future simulations using the CCCma GCM. First, the Land Surface Processes node initially concentrated all its effort on
parameterizing, modifying, and evaluating the Canadian Land Surface Scheme (CLASS). In the last two years several investigators began to work on
modelling various aspects of the terrestrial carbon cycle either by directly incorporating functions into CLASS itself (Arain, Black & Grant), or
by developing parallel models that coupled to CLASS (Roulet). A second effort, through what was referred to as the Carbon (C) node, began to work
on developing the ocean component of the global carbon cycle. This node, primarily due to the loss of its principal investigator (I. Fung), only
got going at the time when the CRN began losing financial support because the funding for climate science in Canada was restructured. When the
Canadian Foundation for Climate and Atmospheric Science (CFCAS) was formed and called for Letters of Intent (LOI), some 10 members of the various
nodes met in Victoria BC, to assess the expertise and desire to develop a Canadian coupled carbon climate model. The result of this meeting was our
submission of a LOI to CFCA, September, 2000.
In January, 2001, our group was informed by CFCAS that they would like to discuss the LOI along with several other initiatives on various aspects of
carbon and climate. This meeting took place in Ottawa on January 29, 2001. Approximately six weeks after the Ottawa meeting we were informed that the
CFCAS would like to see a full proposal from our group and awarded the team $10,000 to assist in the preparation of a proposal. On April 27 and 28, 2001,
the terrestrial carbon node meet in Toronto for a two day workshop to develop their component of the proposal. Similar meetings took place in Victoria
to develop the ocean and atmospheric component of the model. A full proposal workshop was held on May 13, 14 and 15, 2001 in Victoria to bring together
the three nodes and to design the science plan for the integrated modelling effort.
go to top
OVERVIEW OF THE NETWORK
Science Team
Principal Investigators:
Nigel Roulet, Department of Geography & the Centre for Climate and Global Change Research, McGill University, Montreal PQ
Kenneth L. Denman ,Adjunct Academic Appointment at the University of Victoria, Canadian Centre for Climate Modelling and Analysis (CCCma), Meteorological Service of Canada (MSC), University of Victoria, Victoria BC.
Co-investigators:
A. Black, Faculty of Agricultural Sciences, University of British Columbia, Vancouver BC;
R. Grant, Department of Renewable Resources,University of Alberta, Edmonton AB;
G. Boer, G. Flato, and N. McFarlane,Adjunct Academic Appointment at the University of Victoria, CCCma, MSC, University of Victoria, Victoria BC;
A. Arain, School of Geography and Geology, McMaster University, Hamilton ON;
D. Price (adjunct academic appointment at the University of Alberta) , Canadian Forest Service, Edmonton AB.
Collaborators (involvement funded entirely from other sources):
D. Verseghy, Climate Research Branch, MSC, Toronto ON;
V. Arora, CCCma, MSC, University of Victoria, Victoria BC.
Network objectives
1. To develop and test an integrated carbon (C) cycle model including terrestrial, oceanic and atmospheric components for inclusion in the Canadian
Centre for Climate Modelling and Analysis (CCCma) Coupled General Circulation Model (CGCM);
2. To incorporate and test the coupled C cycle model in CGCM simulations aimed at making transient climate change calculations with C as a prognostic
(forecasted) variable;
3. To perform studies of the role of carbon dioxide (CO2) and methane (CH4), the two most important radiatively active greenhouse gases (GHGs) in
regulating the climate system, in order to investigate how the C cycle may respond to a changing climate through dynamic feedbacks.
Targeted area addressed by this proposal
This project addresses the issue of “understanding key climate system processes including greenhouse gas sources and sinks” (from page 4 and 5 of CFCAS’ “Awards Guide”, 2001)
and, therefore, the results
of this work will assist CFCAS meeting some of its objectives of “1. Channel and strengthen Canada’s scientific capacity to address climate change …; 2.
Provide scientific basis for a better understanding of the climate system, climate change,…; 3. Provide the scientific basis for policies to address the
consequences of …., climate change, …” and “Foster collaborative and interdisciplinary approaches to research … climate and climate change.” 4
Brief statement of the scientific problem
Climate and weather are the outputs of a complex dynamical system comprising the atmosphere, land, and oceans that regulates the cycling of heat, water,
radiatively-active gases, and related elements, in particular C, sulphur (S), nitrogen (N), and oxygen. Canada, like a number of other developed nations,
has invested in the ongoing development and improvement of comprehensive coupled climate models. Projections of future climate, from the Canadian and
several other climate models, formed the scientific basis that led to the Kyoto Protocols whereby Canada and other countries agreed to reduce their GHG
emissions, of which the C based gases CO2 and CH4 are the most important after water vapour.
While the CCCma CGCM is continually being improved, it and the other comprehensive coupled models used in the IPCC scientific assessments do not include
C as a prognostic variable. That is, specified global emissions of past, present and future CO2 and CH4 do not lead to the model 'predicting' levels of
atmospheric GHGs and hence, they do not themselves influence simulated climate. Instead, various simplified models are used to convert the emissions
scenarios into projections of atmospheric concentrations of GHGs into CO2 'equivalents' over the next century. These projections are then used to drive
the comprehensive coupled climate models. Since GHG concentrations are prescribed in these simulations, the resulting climate change does not “feedback”
on the rate of C cycling and, hence, on the GHG concentrations themselves.
We have considerable confidence in the data on past and present emissions of GHGs at the global level. However, until the most important GHGs are included
as prognostic variables in comprehensive coupled climate models, these models cannot take the next major step in reducing uncertainties in forecasting
future climate. Such a step would eliminate the conversion from emissions to concentration scenarios via calculations with simpler models and would
instead allow feedbacks between the C cycle and the physical climate system to occur. We propose to develop and test an integrated C cycle model of the
land, ocean and atmosphere, and to implement this model in the CCCma CGCM. This development will enable transient climate calculations, where only
emission scenarios will be specified and the model will forecast
(i) future atmospheric concentrations of the main GHGs - i.e. CO2 and CH4, and
(ii) the subsequent state of the climate, where the important feedbacks linking the C cycle and the climate system are included.
This is a significant and important scientific challenge. The step of moving to full-form systems models that couple C and climate has been referred
to as the “flying leap” (Fung et al., 2001) because of the risks involved and the uncertainties on how exactly to proceed, but it is recognized as
a key and essential step to reducing uncertainty in climate simulation (Sarmiento and Wofsy, 1999). If we consider CO2 alone, present-day global emissions
are approximately 7 Pg-C yr-1 (1 Pg = 1015 g). About half of these emissions remain in the atmosphere, with the remainder absorbed (sequestered) in
the terrestrial biosphere and the oceans in roughly equal proportions. We do not fully understand what factors control these major C sinks, and therefore,
most importantly, we do not know whether they will continue to remove excess C at present-day rates over the next decades and century. Furthermore,
a coupled climate model with an active C cycle will likely yield surprises and serve to identify gaps in our current understanding of feedbacks that
might stabilize or further destabilize the climate system in response to anthropogenic emissions.
We know that the net flux of CO2 of 3-4 Pg C yr-1 into the terrestrial biosphere and the oceans is less than 3% of the gross natural fluxes (roughly150
Pg C yr-1) between the atmosphere. Thus, the challenge is twofold: first, to develop and implement a C model capable of reproducing small differences
between much larger natural fluxes (e.g. the total annual flux to the oceans is ~100 Pg C yr-1 and the return flux to the atmosphere is roughly 97 Pg C
yr-1); and second, to forecast the effect of the possible changes in these small net fluxes in changing climate.
The policy relevance is immediate. If comprehensive climate models with an active C cycle forecast a significant reduction in the ability of the
terrestrial biosphere and/or the oceans to accept future excess GHGs, then current international efforts to manage anthropogenic emissions (e.g.
resulting from the Kyoto Protocol) will be grossly inadequate. Even dramatically greater reductions than those sought by the Kyoto Protocol will
result in concentrations of GHGs double or triple the present concentrations, if stabilization is attained. This model will simulate the climatic
implications of the GHG stabilizations that result from different GHG emission scenarios, and will, therefore, provide direct input to national and
global policies related to impact assessments, adaptation strategies, and the identification of vulnerabilities.
Structure of the network and the importance of individual components in achieving our objectives
The goal of this proposal is to enhance the CCCma CGCM by incorporating in it a representation of the global C cycle.
The terrestrial C cycle will be incorporated through enhancements to the Canadian Land Surface Scheme (CLASS), the land surface model of the CGCM.
The ocean C cycle, including inorganic C and a simplified treatment of the oceanic ecosystem, will be incorporated into the ocean component of the CGCM.
The atmospheric component will be modified to include CO2 and CH4 concentrations as prognostic variables, along with the addition of some simple
atmospheric chemistry. In the resulting model only anthropogenic emissions of CO2, CH4 and other greenhouse gases will be prescribed (Figure 1).
![[figure_1.gif]](pics/proposal1.gif)
Figure 1: General conceptual structure of the proposed GC3M.
The version of CLASS currently used in the CGCM does not include a C component, but several of the Co-Is have incorporated photosynthesis and
stomatal conductance, carbon and nitrogen allocation, and soil carbon dynamics into their local research versions of CLASS. This work will inform
the development of the terrestrial C model for the GC3M, but the current descriptions of ecosystems are far too detailed and require too many variables
and parameters for their use to be computationally feasible for global scale simulations. The challenge is therefore to take this knowledge and develop
some simplified algorithms that can run efficiently for global simulations, yet remain as true as possible to the processes of the terrestrial biosphere.
This portion of the proposed research effort accounts for over half of the resources requested. The main challenge for the ocean carbon model is the
description of the oceanic ecosystem and its interaction with the physical environment. The atmospheric component places the smallest demand on resources,
as it benefits from previous work done on atmospheric tracer transport and separately-funded efforts to include stratospheric and tropospheric chemistry
in the model.
To attain our objectives we are proposing a research network that comprises three nodes representing different themes. The bulk of this proposal describes
the themes in Section 4. The three themes focus on, respectively, the development of a terrestrial carbon model, the development of an ocean carbon model,
and the changes required in the AGCM to accommodate the inputs of CO2 and trace gases from the ocean and terrestrial biosphere. There will be a fourth
network wide theme that focuses on the implementation of the work of the three thematic areas into the CCCma GCM to produce the Canadian Coupled Carbon
Climate Model GC3M)
Training of highly qualified personnel
There is a significant training component to this research network. Over 70% of the funds requested are to go to supporting post-doctoral fellowships,
graduate students, and research assistants as part of cooperative work- study programs. The activities of the personnel to be trained are outlined in
Section 4 and are also detailed in the justification of the budget in Part I of the proposal. It should be recognized that a some of the work planned in
this project is not suitable for training graduate students or post-doctoral fellows, therefore, we have included request for support for several research
assistants/technicians. Their training is also important and the skills attained are very valuable as has been clearly shown by past experience.
go to top
BACKGROUND
The global carbon cycle – climate connection
The global C cycle and climate are intimately related. The 500,000 year record of temperature and the concentrations of CO2 and CH4 are highly correlated
(Petit et al., 1999), but the causal mechanisms that result in this relationship have not been fully elucidated. It is clear from model analysis and the
13C record in sediments that the terrestrial biosphere contained more carbon during the Holocene than during the last glacial maximum. This indicates
that the oceans stored more carbon during the last glacial period than now. There are several hypotheses related to solubility, ocean productivity, and
alkalinity that have been invoked to explain this greater store but a consensus has not yet emerged. The concentrations of CO2 and CH4 were 80 p.p.m.v.
and 400 p.p.b.v. lower during the last glacial maximum than they were during most of the Holocene (Petit et al., 1999). Over the last 11,000 years up
until the industrial revolution, the change in CO2 concentration was approximately 20 p.p.m.v. (Smith et al., 1999; Indermuehle et al., 1999), but over
the past 150 years the concentrations of CO2, CH4, and N2O, the three principal GHGs have increased approximately 85 p.p.m.v., 1045 p.p.b.v., and 44
p.p.b.v, respectively (Ehhalt and Prather, 2001). The current magnitude and rate of change of greenhouse concentrations are, therefore, unprecedented
over the last 500,000 years. A consensus has emerged that a significant portion of the observed 0.6oC increase in temperature over the 150 years can be
attributed to the increase in greenhouse gases, but it is also clear that some of the temperature increase has natural causes (Albritton and Meira Filho,
2001). Based on emissions scenarios for the future, climate simulations project an increase in global average temperature of between 1.4 and 5.8o C by
2100 (Albritton et al., 2001). The projections in the latest contribution of Working Group I to the Third Assessment of the IPCC were based on simulations
using climate models where concentrations of GHGs were prescribed, therefore, they do not include the possible interactions between climate induced change
in the cycling of carbon. A recent coupled carbon climate simulation showed the incorporation of an active carbon cycle can significantly alter the outcome
(Cox et al., 2000). An active carbon cycle resulted in atmospheric concentrations of CO2 that were 250 p.p.m.v. higher than from the uncoupled carbon –
climate simulation, yielding a global mean warming of 5.5oC compared to 4oC. This provides a clear justification of the need to include an active carbon
cycle in the next generation of climate models.
The global carbon cycle that is related to contemporary climate change involves the store and flux of carbon between three reservoirs: the terrestrial
biosphere, the ocean, and the atmosphere. These contain approximately 2100, 750, and 39,800 Gt C, respectively (Schimel, 1995). The terrestrial reservoir
can be divided into living biomass (600 Gt C) and soils (1500 Gt C). Of the ocean carbon pool, approximately 1000 Gt C is dissolved inorganic carbon (DIC)
in the upper ocean and 700 Gt C is dissolved organic carbon (DOC). The majority of the current increase in CO2 and other greenhouse gases is not due to
changes in the carbon cycling among these three reservoirs, but due to the addition of C to the atmosphere from the burning of fossil fuels. This excess
carbon, estimated to be 5.4 and 6.3 Gt C yr-1 for the periods 1980 - 1989 and 1990 - 1999, respectively, is partitioned, unequally, among the atmosphere
, ocean, and terrestrial biosphere (see Prentice et al., 2001 for all values quoted in this paragraph). Of this additional source, 3.2 to 3.3 Gt C yr-1
has remained in the atmosphere increasing the concentration of CO2. The difference between the emissions and the change in the atmospheric reservoir has
gone into the terrestrial biosphere and the ocean. The uptake in the ocean for 1980-1989 and 1990-1999 was –1.9 and –1.7 Gt C yr-1, while the land uptake
was –0.2 and -1.4. The land component has the additional complication that land-use change resulted in a release of between 0.6 and 2.5 Gt C yr-1, with a
central estimate of 1.7 Gt C yr-1, while at the same time there appears to be additional net uptake of carbon in the temperate and boreal forest biomes.
The latter is estimated, as a residual term, to be around –1.9 Gt C yr-1.
Changes in the global carbon cycle can occur as the result of a direct response to changes in the concentration of CO2 and to feedbacks in the cycle due
to climate (Prentice et al., 2001). The uptake of CO2 in the ocean is governed by ocean circulation and chemistry. Ocean uptake increases as the
difference in CO2 partial pressure of the atmosphere and ocean surface increases, but the increase in CO2 in the ocean water decreases the uptake rate
due to a reduction in the buffering capacity of the carbonate system. CO2 also decreases the pH, which can alter calcification, which in turn would
enhance CO2 uptake. The uptake of CO2 in terrestrial plants increases with increasing CO2 through the direct enhancement of photosynthesis and indirectly
through changes in water use efficiency. Temperature increase in the surface ocean reduces the solubility of CO2, which reduces CO2 uptake, but with
warming there is likely to be an increase in stratification reducing CO2 outgassing. Changes in precipitation and temperature affects terrestrial
photosynthesis, autotrophic (plant), and heterotrophic (soil) respiration, but the net effect of these changes is not known.
Other factors, independent of climate change and increasing atmospheric CO2, may contribute further changes in the global carbon cycle. These include
land-use change, which may alter both the carbon pools in the ecosystems and surface albedo. For example, two recent modelling studies have suggested
that while afforestation has the potential to remove CO2 from the atmosphere, this benefit may be negated by reduced albedo, which increases net
absorption of incoming solar radiation (Betts, 2000; Claussen et al., 2001). Secondly, increased nitrogen deposition over the last thirty years may be
increasing productivity of some northern forests, which are typically N deficient (e.g., Kauppi, 1996). Conversely, changes in atmospheric chemistry
(e.g. increased tropospheric ozone) and soil chemistry (e.g. leaching of cations, and increased mobility of aluminum) may reduce forest vigor and make
trees more susceptible to damage, thus reducing forest productivity.
The development of coupled C climate models is in its infancy. There has been considerable effort invested in developing climate sensitive terrestrial
biogeochemical models (TBMs) and dynamic global vegetation models (DGVMs) (Cramer and Field, 1999; Cramer et al, 2000). Ocean C modelling has also
received significant attention though focused primarily on the inorganic C cycle (Doney et al., 2001). However, as indicated above there have been
very few attempts to fully couple these to GCMs.
The development of the Canadian Climate Model
Development
Coupled, meaning ocean – atmosphere, global climate models provide a physically based approach to the study of past, present and future climates.
The current developmental version of the coupled model is the product of a considerable evolutionary history, as indicated in Figure 2, culminating
in the current version, CGCM3, which represents the physical climate system including the atmosphere, ocean, and land surface.
The atmospheric component of CGCM3 is the latest in a series described in Boer et al. (1984a,b) and McFarlane et al. (1992). The current operational
resolution is T47 L32 where dynamic terms are calculated at triangular T47 spectral resolution and the physical terms on a 96x48 grid. The vertical
domain extends to 1 hPa with the thickness of the model’s 32 layers increasing monotonically with height from approximately 100m at the surface to 3
km in the lower stratosphere. While many of the physical processes in this version of the model are similar to those of the earlier versions, new features
include: (1) a new parameterization of convection (Zhang and McFarlane, 1995), (2) improved treatment of radiation, (3) an “optimal’ spectral
representation of topography (Holzer, 1996), (4) revised turbulent transfer coefficients at the surface (Abdella and McFarlane, 1996), (5) a hybrid
moisture variable (Boer, 1995), and (6) the CLASS land surface scheme (Verseghy, 1991, 1993). In the current model, vegetation types and characteristics
are prescribed, as is atmospheric composition.
The ocean component (OGCM3) is based on the National Center for Atmospheric Research (NCAR) community ocean model (NCOM1.3) which is a ‘z-layer’
primitive equation model with a ‘rigid lid’. As currently implemented at the horizontal resolution of 1.8 degrees, there are four ocean grid boxes
underlying each atmospheric grid box. There are 29 levels in the vertical. The model represents sub-grid-scale mixing of tracers via isopycnal
diffusion and the Gent and McWilliams (1990) parameterization of eddy stirring effects. Flato and Boer (2001) illustrate the important changes in
ocean heat (and by implication, tracer) uptake that arise from improvements in the ocean mixing scheme in the coupled climate model. In its standard
form, the ocean component carries only two prognostic tracers, potential temperature and salinity. However, in preparation for implementing an ocean
carbon model, several modifications have been made to allow for an arbitrary number of ‘passive’ tracers - i.e. tracers that do not affect ocean
circulation). This capability has been exploited to include a representation of the inorganic carbon cycle in the model. Work is currently underway
to enhance the vertical and horizontal resolution of the model and to implement the ‘K-profile parameterization’ (KPP) of vertical mixing.
The next step in the evolution of the model is directed towards including a first order representation of physical/biological interactions
with the ocean and land surfaces which affect atmospheric greenhouse gas (GHG) composition and feedback on climate.
Use
Climate model simulations are basic to the study of potential anthropogenic climate change as discussed, for instance by Cubash et al.(2001) in the Third
Assessment Report of the Intergovernmental Panel on Climate Change (IPCC). Results from the Canadian coupled global climate model (CGCM) appear
prominently in several Chapters in the IPCC report (Chapter 8, Assessment; Chapter 9, Projections; Chapter 10, Regional aspects; Chapter 11, Sea
level rise; Chapter 12, Detection) and form the basis of a variety of studies of climate change. (e.g. Flato et al., 2000, Boer et al., 2000ab, Arora
and Boer, 2001ab, Holzer and Boer, 2001).
In addition to generating first order estimates of climate change due to anthropogenic GHG forcing, climate models provide input to a variety of other
studies. Results from the CCCma CGCM have been widely used for impact and other studies via the IPCC Data Distribution Centre and via the CCCma and
Canadian Institute for Climate Studies (CICS) websites (over 12,000 registrants). The model was one of two used in the US National Assessment (2000).
CGCM2 provides the horizontal boundary conditions for the Canadian Regional Climate Model (CRCM2) downscaling calculations (Laprise et al., 1999).
Climate models aim to represent climate from “first principles” that may be expressed in terms of a set of non-linear partial differential equation
(e.g. Boer, 2000). However, a range of assumptions and approximations must be made to progress from the equations to the simulated climate. Uncertainties
arise from many sources, so model results may disagree. It has become increasingly clear from the variety of model intercomparison projects (e.g. CMIP,
Meehl et al., 2000) that there is no single “correct” or even “best” model. Each model can and must contribute to the overall knowledge of the climate
system. In particular, multi-model ensemble methods offer information beyond that provided by any single model. Lambert and Boer (2000) for instance,
show that the ensemble mean model is the “best” model in terms of simulating current climate. Chapter 9 of the IPCC TAR treats climate projections from
an ensemble point of view. The CCCma CGCM both contributes to and benefits from the collection of climate model results that provide new scientific
knowledge as well as practical guidance to decision makers.
The inclusion of biological aspects and the carbon budget, as proposed here would provide additional critical information on the behaviour of ocean and
terrestrial sources and sinks of carbon, on changes in biological productivity, and on the feedbacks to GHG concentrations in the atmosphere and hence
climate. Such information is crucially important both scientifically and for policy development, practically impact assessment and the development of
mitigation strategies.
go to top
THEME AREA – GC3M MODELLING NODES

Science team
Background to terrestrial carbon modelling and theme objectives
Foley et al. (1996) states there are three categories of models developed over the last decade to examine the relationship between processes of
terrestrial ecosystems and climate. The first group is the soil-vegetation-atmosphere-transfer schemes (SVATs) such as BATS (Dickinson et al. 1986)
SiB (Sellers et al., 1986, 1992), LSX (Pollard and Thompson ,1995), and CLASS (Verseghy 1991, 2000; Verseghy et al. 1993). These models attempt to
simulate the biophysical aspects of the exchange of energy, water, and momentum using prescribed vegetation and soil characteristics. The second
group is the “biogeographical” models that attempt to capture the relation between the geographical distribution of plants and climate. An example
of this type of model is BIOME (Prentice et al., 1992; Haxeltine and Prentice, 1996). In these models there is more emphasis on developing functional
plant groupings whose spatial distributions are characterized as a function of key climate variables. An important limitation is that vegetation
distribution is assumed to be in equilibrium with a prescribed (stable) climate. The third group of “biogeochemistry” models, such as TEM (Melillo
et al., 1993, McGuire et al., 1997), CENTURY (Parton et al., 1993), and CASA (Potter et al., 1993; Randerson et al., 1996) have a much more detailed
description of ecosystem biogeochemistry, but usually have less direct coupling with climate. These models simulate the cycling of carbon and other
nutrients through the living biomass and soil organic matter of the ecosystems. They use prescribed vegetation and climate is input either as direct
measurements or from an output of a simulation or observational reanalysis. These models can be used to examine how different climates affect
biogeochemical cycling. Many of the models that fall into the second and third groups have participated in intercomparisons to assess their performance
in estimating global or regional ecosystem net primary productivity (NPP) (e.g. Heimann et al., 1998; VEMAP 1995). The second and third phases of the
Vegetation and Ecosystem Modeling and Analysis Project (VEMAP) have seen the development of spatially explicit dynamic vegetation in some of the models
(S. Runnings pers. com.), but none of these three groups of models provide an explicit link from the ecosystem processes back to climate – the interaction
is one way.
Since the mid-1990s there has been the development of a fourth type of terrestrial biospheric model. This is not really an independent category of models,
but through a convergence of the three groups listed above. Kucharik et al. (2000) refer to this group as dynamic global ecosystem models (DGEMs), but
the label is somewhat arbitrary. In these models the flux of carbon into the ecosystem is tightly coupled to the treatment of the transfer of heat,
water, and momentum. Therefore, they are built on SVATs. DGEMs account for photosynthesis, and plant (autotrophic) respiration, and soil (heterotrophic)
representation, and they have simplistic representations of spatial dynamics of vegetation distribution as a function of climate. If present, natural
disturbances such as fire are typically represented simplistically. Some also account for changes in land-use. Examples of DGEMs are MOSES/TRIFFID
(Cox 2001), IBIS (Kucharik et al., 2000; Foley et al., 1996), Hybrid (Friend et al., 1997; White et al., 2000), and BETHY (Knorr and Heimann, 2001a, 2001b).
These models are being developed with the expectation that they will be dynamically linked to climate models and will, therefore, be used to examine
the distribution and/or the flux of carbon between the atmosphere and the surface as a function of climate and at the same time have the results of the
interaction – i.e. changes in exchange of CO2 influence the concentration of CO2 used in the GCM. MOSES/TRIFFID have been dynamically linked to a
coupled ocean atmospheric general circulation model (Cox et al., 2000). The concentration of CO2 that resulted in a transient climate simulation was
considerably higher as a result of oxidation of carbon from the terrestrial biosphere than that prescribed in uncoupled simulations.
The DGEMs, biogeography and biogeochemistry models have a fully developed schemes for partitioning of the exchanged carbon into living and dead biomass,
and take into account the decomposition of soil organic matter to produce a soil CO2 flux. While these models vary in their level of sophistication,
their treatment of photosynthesis (and as a consequence plant respiration), fall into two groups: using either a gas exchange or light-use efficiency
approach (see below). Most treat soil respiration as a first order function of soil mass. Where the models differ in such things as the number and
assembly of functional plant types, how the canopy is constructed and how this affects radiation transfer, the seasonal dynamics of leaf out and
senescence – i.e. plant phenology, the partitioning of carbon within the plants and soil, and the treatment, or exclusion, of nitrogen as a key nutrient.
The models also vary greatly in how they treat the spatial aspects of vegetation. Some prescribe a fixed vegetation while others incorporate competition
among PFTs and disturbance (more details of some of these aspects are given in Sections 7.1.4.1 through 7.1.4.5).
It is reasonable to ask “With the land surface models listed above why develop another DGEM?”. There are a number of reasons that can
be put forward.
These models generally represent much the same processes, but do so in different ways. A similar argument can be made in the case of the biophysically
based SVATs. The results of the Project Intercomparison of Land-Surface Parameterization Schemes (PILPS) have shown that there are large differences
in how each SVAT performs in standardized evaluation situations. Since the biophysical model is critical to the success of modelling the carbon dynamics
it is important that these models perform well. In these comparisons CLASS, the surface scheme that we will build C-CLASS from, did well (Chen et al.,
1997; Liang et al., 1998; Lohmann et al. 1998; Schlosser et al., 2000). PILPS, like CMIP for the climate models, has shown that there is no correct, or
‘better’ LSP and that each LSP has inherent uncertainties. When SVATs are implemented in GCMs these uncertainties will almost certainly increase given
the range of inputs they will receive from different GCMs (precipitation is a prime example). These uncertainties will multiply when carbon fluxes and
ecosystem dynamics are inserted into the SVAT. This is because the SVAT provides the surface ‘climate’ which drives the ecosystem functions. Also the
seasonal dynamics of the vegetation in a coupled carbon SVAT (C-SVAT) are no longer a simple prescription, but now becomes in part, a function of the
ecosystem itself. For example, leaf area index (LAI) used to estimate surface albedo is a function of leaf biomass, which is grown in the ecosystem
model. Because of the increasing uncertainty and the complexities of linking all these processes, it is highly desirable to have a number of DGEMs that
can couple to climate models so that an ensemble of results can be produced to frame the possible outcomes of climate simulations. As it stands now,
the DGEMs are uniquely coupled to a particular biophysical SVAT (e.g. MOSES/TRIFFID), or they are their own SVAT (e.g. IBIS). In principle, future SVATs
and/or DGEMs could be interchanged among GCMs, though this would require a common SVAT/DGEM – CGCM interface to be developed.
There is ample evidence that the evolution of land surface models for climate studies has benefited immensely from having a number of models developed
simultaneously. Progress has been made through the incorporation of ideas from other groups. In the examination of the current state of DGEMs we have
identified a number of areas where we feel we can contribute to advancement of the field. These ideas form the core of the details described in
Section 4.1.4, but below we present a brief introduction of where we think the representations can be improved.
The current land surface models incorporate photosynthesis mostly through the use of the Farquhar et al. (1980) model for C3 plants and the
Collatz et al. (1992) for C4 plants, or by using a light-use efficiency approach such as that described by Ruimy et al. (1994). C-CLASS (Arain et al.,
in review) has adopted the former. However, the implementation of these photosynthetic models occurs through the parameterization of a relatively
small number of plant functional types (PFTs). Parameterization of the PFTs is limited, in part, by the availability of data, but also due to selection
of those that are viewed as dominant in the terrestrial C cycle. This, we believe has resulted in a bias towards PFTs that are common in warmer regions.
In particular, there are no PFTs that represents plants common to the arctic and subarctic, and wetland ecosystems. In the case of C-CLASS we feel
it is important that the poikilhydric plants, such as lichens and mosses, be represented as a distinct functional plant type. These plants occur in
ecosystems that tend to have low productivity and large stores of carbon (Schlesinger, 1997), located in regions predicted to experience large changes
in climate – and hence they are very important from the perspective of the sink/source of CO2, as well as being potentially large sources of CH4
(see below). Wetlands have not yet been included in any of the global analyses of interactions between climate and carbon dynamics, but they store
between 25% and 35% of the world’s soil carbon (Gorham 1991, 1995). This omission has been clearly recognized (e.g. Melillo et al. 1993; A.
McGuire, pers. com), but wetlands have been ignored because the models have not incorporated the hydrology of organic soils. Organic soil hydraulic
and thermal properties have recently been implemented in CLASS (Letts et al., 2000) and the performance of CLASS for wetlands has significantly
improved (Comer et al., 2000).
Soil respiration in most C–SVATs is represented as a first order function of the mass of soil organic matter, modified to account for the
influence of soil temperature and moisture. However, very recent results from Euroflux show soil respiration is more closely connected to gross
primary production (GPP) than temperature (Janssens et al., 2001). Within an ecosystem soil respiration is correlated with temperature, but differences
among ecosystems are explained by differences in GPP. Janssens et al. (2001) also show that the relationship is strongest for mature forests, and
weaker for disturbed sites and sites that experience droughts. These results indicate a coupling between GPP and soil respiration that cannot be
captured by a first order approximation. Janssens et al. (2001) postulate the connection comes through the ‘priming’ of soil respiration and the
respiration of fast turnover of the litter. The first requires some linking of heterotrophic respiration to production, while the second suggests
an explicit treatment of litter decomposition, rather than adding litter to the first soil layer, as is currently the practice in most models. Some
ability to simulate changes in the recalcitrant nature of soil organic matter could be introduced into C-CLASS. This is particularly relevant for
roots, which effectively “freshen” the soil carbon pool through death and fine root turnover. It is recognized (e.g. see Kucharik et al., 2000 for
self-criticism of IBIS) that the soil component of the models is generally much weaker than the treatment of photosynthesis, even though the soil
component generally plays a dominant role in the climate simulations of Cox et al. (2000). In the treatment of soil temperature and water it has
been found that the number of soil layers, and particularly the number of layers containing roots has a significant effect on the soil water budget
(Martinez et al., 2001). Since respiration and plant production are strongly influenced by soil moisture and temperature, a sensitivity analysis
of the soil water and temperature should be performed to see how such changes affect soil and plant respiration. It is unreasonable to expect SVATs
to have many soil layers, but this analysis would help determine the uncertainties involved in using 1 to 3 layers as is currently the case with
CLASS. The number of soil layers, and the treatment of recalcitrance in particular, are very significant given the current debate over the temperature
response of soil carbon (e.g., Giardini and Ryan, 2000; Davidson et al., 2000).
Nitrogen (N) is an essential nutrient for plant production. It is generally believed that most forested ecosystems are N limited (e.g. Aber et al.,
1989; 1998). At the same time as CO2 concentrations are increasing (with some positive effect on C assimilation), N deposition from intentional
fertilization and air-borne pollution is also increasing. This increase is global (Holland et al., 1999) but in some regions the deposition levels
far exceed natural N fixation. It is not clear whether this extra N is all utilized by plants and/or the microbial community (c.f. Nadelhoffer et
al., 1999), but there is a high retention of deposited N. If soil mineralization increases due to a persistent warming of soils, there is expected
to be a commensurate increase in N mineralization and therefore N availability for plants (Shaver et al. 1992; Oechel et al. 1994). Ecosystem
biogeochemistry models such a TEMs (McGuire et al., 1997) and CENTURY (e.g. Schimel et al., 1996) explicitly treat the coupling between the nitrogen
and carbon cycles. A similar coupling should be attempted for DGEMs (e.g. Friend et al., 1997), which generally assume no N limitation.
The biogeochemical models have estimated global increases in NPP in the order of 10 Gt C yr-1 due to the combined effects of elevated CO2 concentration
and climate simulated for a 2 x CO2 scenario (e.g. Melillo et al., 1993). The first coupled climate – carbon model simulation estimated a net addition
of approximately 100 Gt C to the atmosphere (Cox et al., 2000). This change comes from a combination of approximately 50 Gt C increase in living
biomass offset by an approximately 150 Gt C release of soil carbon by 2100. It has been estimated that land-use changes over the last 140 years have
released a net 130 Gt C to the atmosphere (Houghton et al., 1983; Schimel, 1995); therefore, land-use change contributes a similar magnitude as that
estimated from GHG-induced climate change. Houghton and co-workers (Houghton et al. 1987; Houghton 1996) estimate the current loss of terrestrial C
due to the conversion of forests to other land use is 1 to 2 Gt C yr-1, or between 15 and 25% of the atmospheric burden. It is predicted that alteration
of global biogeochemical cycles attributable to human activities, particularly food production, will increase over the next fifty years (Tilman et al.,
2001). It is also expected that natural disturbances due forest fire and pest out breaks are likely to increase in frequency, particularly in the boreal
ecosystems (Kurz et al. 1995; but see also Flannigan et al. 1998), thereby increasing net release of CO2 to the atmosphere.
While the key objective in coupling climate to the global C cycle is to improve the prediction of changes in atmospheric CO2, eventually the coupling
of climate to surface processes should include some treatment of the exchange of biogenic trace gases. Methane, CH4, is the second-most important
atmospheric GHG affected by human activity. Unlike CO2, human activity dominates the exchange of CH4 in the atmosphere (Fung et al., 1991).
Therefore for climate simulation the emission scernarios are the most critical issue, although it is estimated that approximately 25% of CH4
comes from wetlands. . Interestingly, Walter et al. (2001) show that most of the variability in atmospheric methane results from the interannual
variability in wetland emissions. The two factors that control CH4 emissions from wetlands are the moisture content and soil temperature (Moore
and Roulet, 1995), which are in turn a direct consequence of climate. Since we plan to incorporate wetlands as a land cover type, we can attempt
to model CH4 emissions from wetlands. Several climate-methane models have been developed (e.g Walter et al., 1996), but these models are extremely
detailed in their treatment of process. A simpler approach, based on the detail processes outlined in Walters et al. (1996) and other ecosystem
models such as ecosys, can be developed for C-CLASS.
Theme objectives
The overall objective of the Terrestrial Carbon node is to develop and test a terrestrial carbon model based on CLASS for inclusion in the CCCma CGCM. To be able to attain the overall objective of this theme, several sub-objectives need to be achieved. These objectives are to:
- integrate the work already carried out on surface carbon fluxes at Universities of Alberta, British Columbia, and McGill under the CRN Land Surface
Processes node;
- develop a scheme to account for dynamic carbon allocation between living biomass and soils, particularly the transfers of dead phytomass through
litter fall, and root mortality and exudation;
- include a phenology algorithm to represent the climatically-driven allocation of carbon to the different components of phytomass (e.g. leaf, stem,
and roots); and in particular, to drive leaf area calculations from leaf biomass;
- further develop and improve on the treatment of autotrophic and heterotrophic respiration for both mineral and organic soils;
- introduce a simple representation of the coupling of ecosystem carbon and nitrogen cycles;
- extend the carbon and nitrogen cycles to incorporate the emission and uptake of CH4, and possibly N2O;
- implement a simple representation of the effects of natural and anthropogenic disturbances on the carbon balance of terrestrial ecosystems,
and develop a mechanism to incorporate projections of land use changes among land cover types (e.g. forest to agriculture or reverse, etc.);
- evaluate the terrestrial carbon model against data sets obtained from Canadian and international sources (e.g. BERMS, PCARS, Ameriflux,
Fluxnet-Canada, ABRACOS/LBA, Hapex-Shael, FIFE, etc.); and assess model parameterizations to determine optimal complexity relative to computational
efficiency, and undertake sensitivity analysis; and
- prepare global fields of input variables for vegetation, soils, and disturbance and land-use change statistics.
By the end of Phase I (year 3) we expect to have attained objectives 1 through 5, 9, and the relevant level of evaluation and sensitivity analysis
as outlined in objective 8. We will also have made substantial advances on objectives 6 and 7 by this time. In Phase II we wish to refine the core
of C-CLASS, as well as add the vegetation dynamics and trace gas components. We expect to implement various versions of C-CLASS in the CGCM by the
end of years 1, 3 and 4 in time for simulations to be undertaken by the end of PhaseII.
Development of the Canadian Land Surface Scheme
The Canadian Land Surface Scheme (“CLASS”) was first developed in the late 1980’s as the land surface model for the Canadian GCM (Verseghy 1991, 2000;
Versgehy et al., 1993). Since then its treatment of surface fluxes of energy and moisture has been maintained as state-of-the-art, through ongoing
development undertaken by Dr. Verseghy and various collaborators.
CLASS is basically designed as a so-called “second-generation” land surface scheme. It incorporates an explicit treatment of the vegetation canopy,
including parameterizations of interception of rain and snow, environmental influences on stomatal resistance, and intra-annual variation of canopy
characteristics such as leaf area, height and rooting depth. It treats the snow pack as thermally separate from the underlying soil, and simulates
temporal variations of snow albedo and density. The soil profile is modelled using three horizontal layers, whose thermal and hydrological properties
are allowed to vary. Calculations of surface infiltration, of moisture migration between soil layers, and of freezing and thawing are performed using
physically-based algorithms. Each GCM grid cell may contain up to four sub-areas: vegetation, bare soil, snow under vegetation, and snow over soil.
These four sub-areas develop separate near-surface atmospheric stability profiles and turbulent and radiative fluxes; the fluxes are returned to the GCM
as areal averages.
CLASS has participated in PILPS since its inception in 1992 (see previous section for references). The various PILPS experiments have included simple
model intercomparisons, as well as comparisons against field data. The field datasets utilized to date include a grassland in the Netherlands, an
agricultural area in France, a seasonally snow-covered grassland in Russia, and river basins in the southern U.S. and northern Sweden. CLASS has
consistently performed among the top few models in all of these intercomparisons.
A major initiative aimed at further developing and improving CLASS was carried out under the auspices of the CRN from 1994 to 2001. Under this program,
a LSP node was established involving Dr. Verseghy as principal investigator, and co-investigating groups from twelve universities and three government
laboratories. Each group had the mandate of testing CLASS using field datasets drawn from their own areas of expertise, and improving the algorithms
where weaknesses were found. The work of the node was documented in a special, dedicated issue of the journal Atmosphere-Ocean (Volume 38[1]) which
appeared in 2000.
The advancements made during the period of operation of the CRN LSP node are currently being implemented into a unified version of CLASS, version 3.0,
which is scheduled for completion in mid-2001. The highlights of version 3.0 include improved formulations for soil evaporation and stomatal resistance,
a new module to simulate organic soil hydrology, coupling to the streamflow routing model WATFLOOD, improved representation of atmospheric surface
stability corrections, and refined algorithms for snow properties. Version 3.0 will also include the capability of treating land surface sub-areas
in grid cells using a finer, “mosaic” approach, rather than the lumped-canopy, which has been used to date. This will enable the model to take advantage
of new, high-resolution global land cover and soils databases. Whereas in the mid-1980’s the best global land cover datasets were only available at
resolutions of 1o x 1o, advances in satellite technology and inversion algorithms have now resulted in resolutions of 1 km x 1 km. How to represent
this more detailed information on the land surface within GCM grid cells, which are still on the order of 2-4 degrees in latitude and longitude, will
be a topic of ongoing research for some years to come.
Within the CRN LSP node, in addition to the refinement of the energy and moisture fluxes and budget calculations done within CLASS, three
co-investigating groups did some preliminary work on incorporating a C cycle into CLASS. The UBC group concentrated on developing a “top-down”
approach towards the representation of photosynthesis, net C uptake and respiration in vegetation. The group at the University of Alberta worked
from a “bottom-up” approach, essentially “mining” the comprehensive ecosystem model ecosys, and adapting algorithms drawn from it for use in CLASS.
The group at McGill University devoted particular attention to C cycling in organic soils and peatlands. The present proposal builds on this work,
and aims to extend it to a full-fledged, interactive treatment of carbon in CLASS. As in the case of the energy and moisture cycles, consideration
will also have to be given to how to deal with surface heterogeneity, as reported in high-resolution global datasets, using a limited number of plant
functional types with lumped parameters.
Outline of the proposed structure of the terrestrial carbon model
The proposed dynamic terrestrial carbon model will include representation of canopy physiology (photosynthesis, conductance), plant phenology (leaf out,
senescence), terrestrial C balance (plant and soil respiration, ecosystem productivity) and C and N dynamics (allocation, plant and soil N cycle and
competition among plant functional types (PFTs) (Figure 2). Version 3.0 of CLASS will provide an improved formulation of land surface physics. These
components of the model will be organized in a logical modular structure and will operate at several time steps ranging from 20 minutes to one year.
Use of variable time steps will allow a logical coupling of biophysical, physiological and ecological processes operating at different time scales.The
terrestrial model will be driven using forcing variables from the GCM (or observed meteorological variables in the offline mode) including downwelling
shortwave radiation, net or downwelling longwave radiation, precipitation, air temperature, wind speed, atmospheric pressure and specific humidity. Values
of key prescribed physiological and phenological parameters, which vary with C3 and C4 PFTs such as coefficients related to intercellular CO2
concentration, stomatal sensitivity to vapour pressure deficit, quantum efficiency for CO2 uptake, etc. will be defined in the form of a lookup table.

Figure 2: The main components and their time steps in the terrestrial carbon model
Carbon fluxes: photosynthesis, autotrophic and heterotrophic respiration
In the previous work with the CRN we developed a realistic top-down single-layer model of CO2 uptake and loss by incorporating sunlit- and shaded-leaf
photosynthesis, canopy conductance and plant and soil respiration (autotrophic and heterotrophic respiration) parameterizations in C-CLASS (Arain et al.,
submitted). The C-CLASS "big leaf" is split into a sunlit leaf and a shaded leaf to prevent the overestimation of canopy photosynthesis in big-leaf models
that results from the non-linear quantum response of leaf photosynthesis. The fraction of sunlit leaf area is calculated using the extinction coefficient for solar radiation penetrating the canopy. Model equations are solved iteratively for each of the sunlit and shaded big leaves using absorbed photosynthetically active radiation (PAR) to give the respective photosynthesis rates and stomatal conductances. The bulk stomatal conductances of the sunlit and shaded leaves are calculated separately using a modified version of the Ball-Woodrow-Berry-Leuning formulation (Wang and Leuning, 1998). This relates stomatal conductance to CO2 assimilation rate, vapour pressure deficit, CO2 concentration at the leaf surface and soil water stress. Sunlit and shaded photosynthesis rates and conductances are added at the end of each time step to calculate canopy values based on respective fractional leaf areas. The model takes 10-20 iterations for leaf temperature to reach equilibrium during each time step, which makes it suitable for use in the Canadian regional climate model (CRCM) as well as CGCM.
Leaf photosynthesis, which is the minimum of the enzyme (Rubisco) and the light-limited carboxylation capacities for CO2 uptake, is based on the work
done by Farquhar et al. (1980) adopted in the single canopy layer photosynthesis model of Wang and Leuning (1998). The supply and demand equations for
carbon assimilation in the photosynthesis model are solved by adjusting the concentration of CO2 in the intercellular air spaces of the leaf. The model
also takes into account the prescribed vertical distribution of nitrogen in the canopy to calculate gross photosynthesis, but in the future we would like
to have this concentration computed from an active nitrogen cycle (see Section 4.1.4.3). The values of parameters for the big leaves are scaled up
separately over all the sunlit and shaded leaves by taking into account distribution leaf nitrogen and PAR within a canopy. In our current model, all
the vegetation is assumed to have C3 metabolism. Photosynthesis for C4 plants, which are mainly tropically grasses, will be included in the proposed
terrestrial model and based on work done by Collatz et al. (1992). In the current C-CLASS formulation, a key photosynthesis parameter, the maximum
carboxylation capacity of Rubisco (Vcmax) is prescribed as a constant value for different vegetation types. Rubisco catalyzes the first step of carbon
assimilation by combining CO2 with the ribulose bisphosphate. Its concentration limits the rate of photosynthesis under light saturated conditions.
Different studies (e.g., Field and Mooney, 1986, Wilson et al., 2000) have shown that Vcmax has a strong relationship with leaf N content. They showed
that leaves with the highest nitrogen on a mass basis also have the highest rates of photosynthesis. Thinner leaves generally have a larger carbon to
nitrogen (C/N) ratio. Therefore leaf C/N ratio could be used as an alternative to Vcmax. In nature leaf nitrogen content is not fixed but responds to
the overall plant-soil nitrogen cycling processes, which are strongly connected to various climate and soil variables such as temperature, precipitation
and soil moisture. In this proposal we intend to derive photosynthetic capacities for all PFTs from nitrogen cycling so that they can interact with
climate and soil processes. Because canopy conductance is related to photosynthesis, the N derived Vcmax values will link canopy assimilation and
transpiration to variations in N availability.
A simple model of autotrophic (plant) and heterotrophic (microbial) respiration is also incorporated in C-CLASS to simulate net ecosystem productivity
(NEP). Autotrophic respiration is broken into maintenance and growth respiration (Ryan et al., 1997). Growth respiration is assumed to be 30% of net
photosynthesis as suggested by Poorter and Villar (1997) and Amthor (1984). Leaf maintenance respiration is assumed to be proportional to Vcmax and
nighttime values are assumed similar to daytime values. Stem and root maintenance respiration depends on biomass, base respiration rate at a reference
temperature and a canopy temperature sensitivity function based on a Q10 coefficient for the respective biomass pool (Bonan, 1995). Maintenance respiration
is reduced by 50% out side the growing season. Heterotrophic respiration is calculated from the soil carbon pool size and the soil respiration rate at
a reference temperature based on work done by Bunnell et al.(1977) and Bonan (1991). The temperature dependence is incorporated using the temperature
of the upper soil layer and a Q10 coefficient. A moisture factor reduces microbial respiration when the soil begins to dry out. Net primary productivity
(NPP) is calculated by subtracting autotrophic respiration from gross primary productivity (GPP). Net ecosystem productivity (NEP) – i.e. the
sequestration of atmospheric CO2 by the ecosystem, is calculated by subtracting both autotrophic and heterotrophic respiration from GPP.
Although we used one root and one soil carbon pool to calculate plant and microbial respiration in Arain et al. (submitted), we also tested a respiration
model which explicitly accounts for root biomass and soil carbon in the three CLASS soil layers and uses their temperature and moisture content values.
We include more than three soil layers to improve the representation of below-ground respiration processes. We will initially focus on the first order
approach, but we intend to develop a simple process-based soil CO2 efflux model for C-CLASS. We have found that the model must accurately describe the
dependence of CO2 source strength on soil temperature, water content, porosity, and amount and type of soil organic matter. This model development work
will help to determine the relative importance of soils in the net CO2 exchange from each PFT. It will also relate soil respiration to the soil N cycle
by including organic and mineral N pools. A root nutrient uptake model will also be developed by taking into account N mineralization, nitrification,
leaching and fertilization. We also intend to use our measurements of soil CO2 efflux (using automated chambers), CO2 concentration profiles and
environmental variables at four forest sites across Canada (the Douglas-fir site on Vancouver Island and the three boreal sites in Saskatchewan), and a
wetland site (the PCARS Mer Bleue in Ontario) to develop and validate the soil respiration model.
Carbon stocks: Living biomass and soils
In C-CLASS carbon assimilated through photosynthesis is allocated to leaf, stem, and roots using constant allocation ratios (Gower et al.1997; Steele et
al.1997). Simple parameterizations to account for prescribed losses of carbon due to drought, frost damage, fires, insect attack, wind throw and harvest
are also included (Dickinson et al.1998) and are used to update the soil C pool. Carbon in the plant and soil pools is updated at the end of each half-hour
, but C can be accumulated on a longer time step such as one day. These parameterizations need further testing for different vegetation types and are not
reported in Arain et al. (submitted). The current treatment of C allocation in C-CLASS is inadequate because allocation to stem and roots changes with
age of vegetation. During drought and nutrient stress conditions plant root allocation may also increase. The explicit representation of age-classes
in forest PFTs will allow these age effects to be investigated (see Section 4.1.4.4).
In the current modelling initiative we intend to develop a fully interactive dynamic carbon model to define the state of soil and plant C pools and to
simulate climate-vegetation feedback. Net primary productivity will be allocated to grow PFTs in each gridcell (Figure 3). PFT structural parameters
(e.g. canopy height, LAI) and land surface parameters (e.g. roughness height, albedo, cover area) will be updated so that changes in the biophysical
properties of the land surface and changes in PFT carbon stock can be coupled with the atmosphere. PFT growth will also include expansion into new areas
and competition for resources (soil moisture and nutrients) with other PFTs. We also wish to include the potential to reallocate C and N at senescence
and an explicit treatment of that year’s litterfall.
Inclusion of the terrestrial nitrogen cycle
Only a few land surface schemes intended for use with GCMs currently represent ecosystem N transformations (e.g. Friend et al., 1999). These models are
therefore generally unable to fully address several key questions about terrestrial C exchange during climate change.
To represent N transformations in C-CLASS, each state variable for plant and soil C described in section 4.1.4.2 will be linked to one for N.
These variables will be initialized from C:N ratios provided for each soil layer and PFT, but will be determined, thereafter, by the N transformations
in the model. Net mineralization (or immobilization) of N from decomposition of plant litter, microbial biomass and soil organic C (SOC) will be
calculated from the first order decomposition of organic matter divided by the current C:N ratio of the substrate state variables (e.g. Powlson et
al., 1996). The partitioning of mineralization products among biomass, SOC and mineral fractions will be determined by contents of litter lignin and
soil clay (e.g. Parton et al., 1987). This partitioning will add (or remove) N from a mineral ammonium pool. This pool will also be a source/product
of cation exchange and a source of nitrification (first order function from Grant, 1994), the product of which will contribute to a nitrate pool.
Ammonium and nitrate pools will exchange N among soil layers through vertical convective-dispersive transport, and nitrate pools will lose N through
first order denitrification (based on Grant, 1991). These pools in the upper soil layer will also receive depositions of atmospheric N. This will be
a prescribed input field based on Holland et al. (1999). All first order parameters will be multiplied by dimensionless reaction-specific functions of
soil temperature and water content as described in section 4.1.4.1.
Both ammonium and nitrate pools will be sources for root uptake by coupling radial convective-dispersive transfer from soil to root surfaces with
Michaelis-Menten active uptake from root surfaces into root N storage pools (Grant, 1998a). Rates of active uptake will be determined by soil temperature
and root surface area, and a feedback mechanism to suppress uptake when storage N is excessive based on C:N ratios in root storage pools
(Itoh and Barber, 1983). Because the same ammonium and nitrate pools are involved in atmospheric deposition, soil transformations and root uptake,
C-CLASS will be able to address questions related the role of N in the assimilation of elevated CO2.
Root C and N storage pools will be Michaelis-Menten substrates for root growth. These pools will also be sources/sinks for root-shoot exchange
(concentration gradient) with storage pools for C and N in leaf and stem. These pools will be Michaelis-Menten substrates for leaf and stem growth.
The C:N ratios of leaf, stem and root growth will be determined by those of the storage pools (Grant et al., 2001b). The resulting C:N ratio of the
leaf will determine its CO2 fixation rate through the use of N-specific parameters (e.g. Evans, 1989) for carboxylation and electron transfer in the CO2
fixation algorithm (Farquhar et al., 1980) described in section 4.1.4.1. A feedback mechanism to suppress CO2 fixation when N is deficient (i.e. Rubisco
inactivation as in Stitt, 1991) will be derived from C:N ratios of leaf storage pools. Because the leaf storage pool exchanges C and N with root storage
pools, and because it influences CO2 fixation through its effects on leaf C:N ratios and Rubisco activation, C-CLASS will be able to address the natural N
constraints on GPP, and to what extent ecosystems will respond to increased N deposition.
C and N state variables will be added to the soil C subroutine to represent non-symbiotic N2-fixing microbial biomass. The C:N ratio of these variables
will be used to determine rates at which C is oxidized and N2 fixed, again according to the stoichiometry of Schubert (1982). Because both symbiotic and
non-symbiotic N2 fixation will be driven by the oxidation of CO2 fixation products in the plant and soil respectively, C-CLASS will be able to address
the third question. Because the products of symbiotic and non-symbiotic N2 fixation are made available for plant and microbial growth, C-CLASS will be
able to examine the feedback between production and N mineralization (as demonstrated in Grant et al., 2001a).
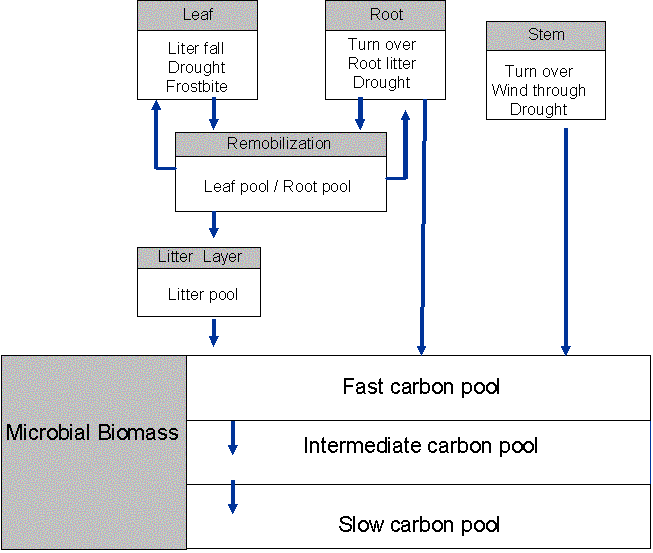
Figure 3: The location of carbon and nitrogen among above and below ground pools.
Plant phenology
In C-CLASS leaf area index (LAI) is used to calculate canopy photosynthesis and to scale-up model parameters for sunlit and shaded big leaves. In the
results reported in Arain el al. (submitted) observed LAI values were used to derive the time series in the off-line model. However, we have tested a
simple algorithm similar to that of Kucharik et at. (2000) for determining the leaf emergence date for deciduous vegetation by accumulating growing
degree-days above a threshold air temperature of 5oC (Arain et al., submitted). This gave excellent results in estimating the date of leaf emergence for
the BERMS old aspen forest for the last six years (Barr et al., 2000; Black et al., 2000). After leaf initiation, LAI increases at a prescribed fixed rate
and full canopy development is completed in one month for deciduous trees and in two month for conifer trees (as in the original CLASS, Verseghy et al.,
1993; 2000). The model’s leaf senescence starts when the five-day daily mean air temperature falls below 13 oC and leaf fall is also completed in one
month for deciduous trees. We will test and refine this approach during the first phase of this research by developing an interactive LAI formulation
in which a fraction of assimilated carbon would be allocated to leaves. This fraction will be defined by an exponential function, which would be
constrained by maximum and minimum LAIs assigned to each PFT. Allocated biomass would be converted to LAI using constant values for specific leaf area
for each PFT. These values would be linked to leaf C/N ratios. During the second phase, we plan to evaluate the physiologically-based approaches that
account for (1) the reduction in the GDD requirement for leaf emergence as a result of an increase in chilling days (Murray et al., 1989), (2) the effect
of duration of photoperiod on leaf emergence, and (3) the effect of water stress on leaf expansion and senescence.
Seasonal changes in leaf area and physiological activity of plants have a significant impact on energy, water and carbon exchanges. In boreal aspen forests,
sensible heat flux is highest during the pre-leaf period, while latent heat flux dominates when leaves are present (Blanken et al 1997). Hogg et al (2000)
postulated that leaf phenology feedbacks contribute significantly to the distinctive seasonal patterns of temperature and precipitation in the southern
boreal region of North America. We have found that the interannual differences in spring NEP in a boreal aspen forest (BERMS Old Aspen site) closely
matched leaf emergence and expansion (Barr et al 2000). The interaction between climate and phenology appears to be a key determinant of global atmospheric
CO2 concentrations (Kindermann et al 1996).
Both empirical and physiological models have been used to estimate leaf emergence. The former are based on the assumption that budburst occurs when
accumulated growing degree days exceed a certain threshold (e.g. Botta et al 2000). The latter attempt to account for the effects of factors such as
duration of photoperiod and winter chilling conditions on the temperature dependency of leaf emergence (White et al 1997, Hänninen 1994). While more
detailed models are desirable, they do not appear to produce better results when applied to a wide range of biomes (Hunter and Lechowicz 1992).
go to top
Dynamic vegetation
Landscape-scale ecosystem processes can be separated into disturbances (both natural and anthropogenic), where the existing vegetation is generally replaced
by similar land cover and land-use change (LUC), when human pressures or management contribute to a persistent alteration in land cover (e.g., from forest
to agriculture). Both processes can contribute relatively rapid and significant changes in ecosystem C storage, superimposed on the slower dynamics
attributable to vegetation growth, litterfall, decomposition and soil carbon accumulation, discussed in Sections 4.1.4.1 and 4.1.4.2. It is now widely
recognized that these landscape scale processes contribute significantly to the terrestrial C balance, although algorithms for their inclusion in global
scale models are only just being developed (e.g., Price and Apps 1996; Schimel et al. 1997; Kurz and Apps 1999).
Globally, human-caused LUC in less-developed regions is likely to be the most significant influence on ecosystem C storage (e.g., Houghton et al. 2000;
Ramankutty and Foley 1999a; Caspersen et al. 2000; Tilman et al., 2001). In addition, deforestation in regions such as the Amazon basin, is likely to
have important consequences for both regional and global cycling of water as well as carbon (see Lettau et al. 1979; Costa and Foley 1997, 2000; Claussen
et al. 2001).
Tropical deforestation effects on global C balance may be offset, both by increases in forested areas at higher latitudes, and because portions of
deforested regions are often allowed to revert back to forest cover within a few years (see Price and Apps, 1996). On the other hand, the establishment
of forest plantations on marginal agricultural land to sequester atmospheric CO2 as an offset to emissions (e.g., Chen et al., 2000b) may also be
problematic. Simulations using simplistic coupling of vegetation to global climate models suggest that large-scale afforestation (particularly with
coniferous species) would cause reductions in albedo that could offset the reductions in warming achieved through CO2 sequestration (Betts 2000; Claussen
et al. 2001). Clearly this is an important feedback that deserves to be investigated more fully before countries such as Canada venture into major
afforestation programs to manage GHG emissions.
In boreal forest regions, a warmer and drier climate would likely contribute to greater losses of forest due to fires and insect pests (as in Canada and
parts of Europe and Asia; e.g. Kurz et al. 1995). Some studies using current GCMs suggest that changes in fire frequency will vary appreciably, possibly
even decreasing in some regions (e.g., Flannigan et al. 1998). Such changes in disturbance regimes (frequency and intensity), combined with the effects of
climate change on forest productivity and decomposition discussed earlier, could trigger profound changes in forest distribution and composition with
further feedbacks to global climate. E.g., surface albedo may change as discussed by Bonan et al. (1992) and Cox et al. (2000). Other more subtle effects
may occur through changes in leaf area and canopy conductance resulting from shifts in vegetation composition, e.g. between coniferous and deciduous forest
(Betts and Ball, 1997; Hogg et al., 2000). Even where species composition does not actually change, an increase in disturbance frequency will generally
reduce the mean C storage density in vegetation and soils—with potentially important consequences for atmospheric GHG composition and ecosystem C
management strategies (Kurz et al, 1999; J. Chen et al., 2000a; W. Chen et al. 2000b).
We propose to simulate both LUC and disturbance processes in C-CLASS. Implementation of both sets of processes will require related, though somewhat
different, approaches.
Simulation of disturbances will follow an approach currently being tested in the IBIS 2.1 model by Price et al. (manuscript in prep., provisionally
authored by Ramankutty et al., see also Figure 4). The “Age-Class Management Engine” (ACME) is a relatively efficient means for tracking changes in
land area fractions of GCM gridcells. Upper canopy PFTs are split into distinct age classes (or cohorts), and C allocation is tracked for each
PFT/age-class combination. Upper canopy PFTs are characterized by the presence of trees, and typically represent tropical, temperate and boreal
forest types. In contrast, lower canopy PFTs are assumed to have no age-class structure, and a disturbance is assumed to cause no significant effect
on annual primary production and C storage. Increased removal of upper canopy leaf area due to more frequent simulated disturbance will give understory
PFTs a competitive advantage, however, that will tend to cause forest to be replaced by grassland (e.g. see the work of Daly et al. 2000).
For each gridcell and each PFT, a set of defining equations captures the recruitment of land area from one age class to the next at intervals corresponding
to the width of a single age class (nominally one year, though it can be wider). A second algorithm allocates to each age-class, the products of NPP
derived from canopy-level photosynthesis and respiration calculations (performed for each PFT as homogenized canopies). This allows changes in C storage
to be tracked with age (and as climate changes occur). Although adjustments in C densities are determined internally, they are written to an external data
file at the end of each annual (or longer) update. Rates of disturbance will initially be prescribed from global statistics and/or simple empirical
relationships with observed climate determined off-line. There will be provision however to extend this so that disturbance probabilities can be estimated
from the forcing climate and (possibly) simple indices of susceptibility derived from the simulated vegetation.
LUC will be represented more as a prescribed rate of change, based on a combination of historical data and future projections (e.g., UN statistics and
other data sets, such as that compiled by Ramankutty and Foley, 1999a). To do this it will first be necessary to identify distinct land-use types. Based
on other work (e.g., with the IBIS model by Ramankutty and Foley, 1999b), it is likely that four distinct types will be needed: urban, agricultural,
natural ecosystems and wetlands (see Figure 4A). The separation between “natural ecosystems” and “wetlands” is needed because it will be very difficult
to simulate the hydrological conditions that lead to wetland formation and destruction at the gridcell resolutions used in current and anticipated
near-future GCMs. Hence it is easier to conceive of “natural ecosystems” which allow for dynamic shifts among tundra, forests, grasslands and desert
vegetation, juxtaposed with fixed areas of wetlands to represent the long-term uptake and release of CO2 and methane at the gridcell scale. The wetland
distribution will be obtained from the IGBP-DIS Wetland project.
Figure 4 provides a diagram of the proposed implementation of disturbances and land-use change in the GC3M. In Figure 4A each grid cell is initially
divided into several aggregated area fractions based on local data, or historical land use statistics. Land cover or land use types may be either static
(wetlands, lakes and urban areas) or dynamic (“natural ecosystems” and agricultural land). Lakes and wetlands would be exposed to climatic forcing and
could respond accordingly (e.g. increased evaporation and faster decomposition under warmer, drier conditions), but their area fractions would be assumed
not to change appreciably during model runs, and changes would be prescribed.
The area fractions allocated to agricultural and “natural ecosystems”, on the other hand, would be allowed to change in response to prescribed scenarios
of land-use, updated on annual or longer timesteps, on the assumption that changes in the agricultural land fraction are exactly matched by equal and
opposite changes in the “natural ecosystem” cover fraction. Figure 4B shows the area fraction allocated to natural ecosystems would support a number of
competing PFTs. Each PFT would be parameterized following the approaches of Kucharik et al. (2000) and Haxeltine and Prentice (1996), so that changes in
climate affecting the grid cell would result in simulated shifts in vegetation composition and other attributes (such as NPP and biomass C density). As
in IBIS, changes in PFT dominance could take several simulated years, or even decades, depending on the rate of simulated change and the adaptability of
the individual PFTs resulting from their parameterizations. Figure 4C, illustrates the case of the forest PFTs, where the effects of disturbance would
also be represented by including a simplistic representation of age-class dynamics. The main reasons for this are that simulated biomass carbon pools will
behave more realistically, and that rapid changes in vegetation can occur when stand-replacing disturbances are represented.
Obtaining global disturbance statistics for “natural ecosystems” will require some concerted effort, even as a first approximation. There are reasonable
data sets available for Canada (e.g., Kurz and Apps 1999; Stocks et al. Canadian Forest Service, pers. comm.) and being worked on for Russia. Comparable
data for the tropics and temperate regions may be more difficult to locate. Arguably, natural disturbance regimes for temperate forests are less intense
than in boreal regions (though where forest harvesting is significant, large-scale statistics should be available). For tropical regions, disturbances may
be less important than land-use change.
Kurz and Apps (1999) described a set of disturbance C transfer coefficients used to track the typical proportions of biomass carbon transferred to other C
pools (i.e., litter and soil) or released to the atmosphere. As part of the global disturbance data set, we would also compile a set of transfer
coefficients. These would be derived from data available from national and regional sources world-wide, and/or educated guesses where data are
unavailable.
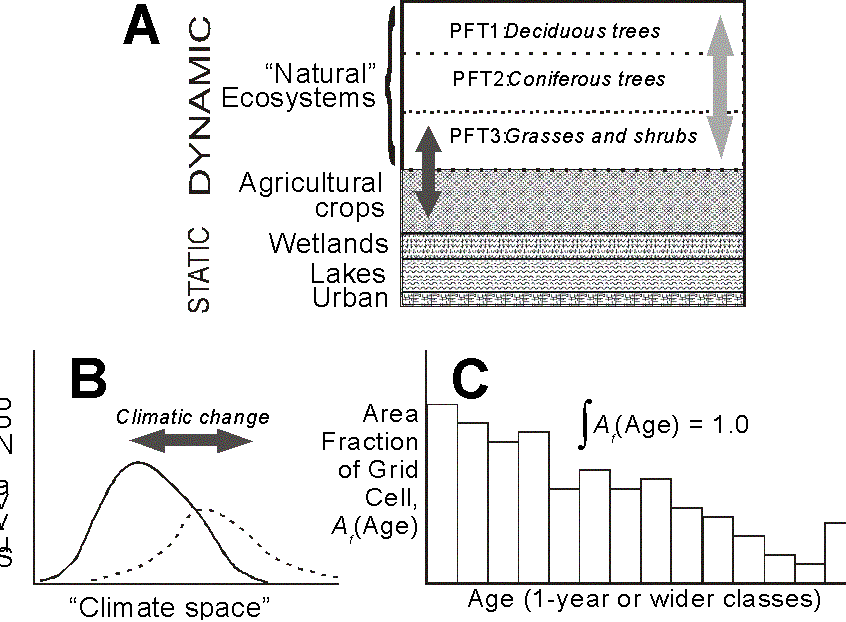
Figure 4: Three issues related to the introduction of the spatial dynamics of vegetation. A. Representation of how landcover and PFTs would be distributed
in a gridcell. B. The notion of the ‘climate space’ of a PFT. C. Age-class distribution.
Incorporation of biogenic trace gases
About 45% of the increase in radiative forcing from greenhouse gases are attributed to increases in methane and nitrous oxide, both of which can be emitted
from terrestrial ecosystems. These gases are not represented in current land surface schemes, so these schemes cannot address important questions about
changes in atmospheric composition during climate change.
To represent methane generation in C-CLASS, state variables will be added to the soil C subroutine to represent a heterotrophic microbial
population that oxidizes and reduces litterfall and soil C to CO2 and CH4 respectively, based on Grant (1998), Cao et al. (1995), and Frolking et
al. (in prep.). The model functions for oxidation and reduction will be modified by temperature based on Dunfield et al. (1993) and on Frolking and
Crill (1994), and by soil water content based on Martikainen et al. (1993), Fechner and Hemond (1992), and Moore and Roulet (1995). The litterfall C
substrate used for methane generation in the model will effectively couple methane emissions to net primary productivity, as oberved by Aselmann and
Crutzen (1990).
Products of methane generation will undergo vertical convective-dispersive transport in aqueous and gaseous phases as in Grant (1998;1999).
State variables will be added to the soil C subroutine to represent an autotrophic microbial population that oxidizes these products to CO2 based on
Grant (1999). The model function for oxidation will be modified by temperature based on Dunfield et al.(1993), King and Adamsen (1992) and Lessard et al.
(1994), and by soil water content based on Adamsen and King (1993) and Lessard et al. (1994). Methane emissions from the soil surface in the model will
therefore be the net of generation and oxidation.
The denitrification scheme described in Section 4.1.4.3 will be resolved into two sequential functions, based on the more detailed reduction
sequence modelled in Grant and Pattey (1999). The substrates of the first will be nitrate and litterfall+soil C (Smid and Beauchamp, 1976), and its
products will be nitrous oxide and CO2. The substrates of the second will be nitrous oxide and litterfall+soil C, and its products will be N2 and CO2.
The functions will be modified by soil temperatures (Bremner and Shaw, 1958; Nommik, 1956) and water contents (Allison et al., 1960; Molina et al., 1985).
The nitrification scheme described in Section 4.1.4.3 will be resolved into two parallel functions based on Grant (1995). The substrate for both functions
will be ammonium, but the products will be nitrate and nitrous oxide. The functions will be based on the stoichiometry of Poth and Focht (1985), and will
be modified by soil temperatures and water contents according to Goodroad and Keeney (1984). N2O emissions in C-CLASS will thus be driven by both
nitrification and denitrification.
Model Parameterization for specific ecosystem types
There will be a considerable number of parameters and data sets required to initialize C-CLASS. We will use the various global soils and vegetation data
sets (1 x 1 km2, 1 x 1o) to construct the spatial distribution of the PFTs for our land cover types. Land-use inputs will be constructed from the work of
IGBP-LUCC. Global nitrogen deposition will come from Holland et al. (1999). We anticipate a considerable effort will be required to assemble this data but
we intend to exploit as much as possible work that has been done in this area already.
Off-line evaluation of the terrestrial carbon model
The initial evaluation of C-CLASS compared modelled results to measured energy and carbon flux data for 6 years for a mature aspen forest and 4 years for a
mature black spruce forest (BERMS sites) in Saskatchewan’s southern boreal zone. The model produced favorable results in simulating stand level carbon
exchange and captured the sensitivity of net ecosystem productivity (NEP) to seasonal and annual climate variability (Arain et al., submitted). C-CLASS
will be tested for all of the major global ecosystems for timescales ranging from hours to multiple-years where observational data are available. Data
sets will be provided through collaborative relationships with Canadian scientists, the Meteorological Service of Canada, international collaborative
efforts (ABRACOS, LBA, FIFE, Hapex-Sahel, Monsoon90, global Fluxnet sites) and will also benefit from the “Fluxnet-Canada Towers Programs” (NSERC/CFCAS
proposal, PI. Hank Margolis).
C-CLASS will be evaluated using the following methodology:
A) Diurnal, Annual, and Interannual Simulations
We will assess the ability of the model to produce realistic diurnal and annual courses of soil temperature and moisture, radiation and energy balance
components, canopy temperature and conductance, net primary productivity (NPP), net ecosystem CO2 exchange (NEE), and net biome productivity (NBP).
Long-term (multi-year) data sets (e.g. BERMS, Camp Borden, PCARS, AmeriFlux, Euroflux, and Mediflux sites), will be used to evaluate the ability of
C-CLASS to account for changes in ecosystem structure (succession and carbon pools) and climatic variability.
B) Carbon Component Flux Analysis
Evaluation will include the use of data sets describing the carbon flux components for selected ecosystems of different PFTs. It is inadequate to evaluate
the model’s performance using only above-canopy fluxes obtained from the eddy correlation method. Compensating errors in model output can often give false
confidence in model reliability. We plan to use biotic chamber data sets (root, soil, stem, leaf CO2 fluxes) obtained at sites where long term above-canopy
flux measurements have been made simultaneously. The BOREAS data archive (BORIS) provides an excellent source of such data (see BOREAS Special Issues J
Geophys Res 102 (D24) 1997 and 104 (D22) 1999). For example, Lavigne et al (1998) compared scaled-up chamber measurements of soil, woody tissue and folia
respiration with nighttime eddy correlation flux measurements in six boreal conifer stands. There are currently 3 sites in Canada (BERMS and Douglas-fir
Vancouver Island) where continuous automated biotic chamber measurements are being made. A fourth, PCARS Mer Bleue, is planned as part of Fluxnet – Canada.
In the case of forests, we will also use eddy correlation measurements to obtain spatially-averaged understory and soil fluxes for validation of C-CLASS
processes including photosynthesis and respiration (Black et al 1996; Blanken et al 1998; Janssens et al 2001b). These data will be invaluable to the
testing of C-CLASS processes. Similar data sets will be obtained from collaborative research efforts with AmeriFlux and Euroflux scientists.
C) Ecosystem Model Comparison and Sensitivity Analysis
C-CLASS simulations of C and energy, vegetation distribution, and carbon pool size will be compared to the output from a number of other ecosystem models
including ecosys, IBIS, and Moses-TRIFFID. Significant differences in the output between models will be investigated by examining the sensitivity of the
underlying processes in each of the models. Replacement of specific algorithms and parameters in C-CLASS, which result in a reduction of sensitivity and
improved model performance should reduce model uncertainty. The model inter-comparison is thus expected to achieve improvements in the accuracy and
consistency of C-CLASS within the precision of the measured data.
Currently there are two model intercomparison studies we would wish to participate in once we have an operational terrestrial carbon model.
The first is to continue the work with PILPS. This will be done through the ongoing activities of DV. The second is to begin to participate in the
Ecosystem Model/Data Intercomparison (EMDI). This study is being run through the Global Analysis, Integration and Modelling Task Force of IGBP. We have
contacted the program manager, Kathy Hibbard, but it would be at least two years before we would be ready for our first involvement.
D) Carbon Budget Uncertainty Analysis
The uncertainty in the annual carbon budget for the major ecosystems will be examined by performing a number of Monte Carlo simulations. This technique
selects random parameters for a number of selected processes from “parameter space”. The exploration of parameter space (random selection of parameters)
will help us determine the uncertainty of annual carbon flux estimates and direct future field studies for improving the parameterization of C-CLASS
processes.
E) Sensitivity analysis of C-CLASS parameters using multicriteria methods
An automated multicriteria calibration procedure will be used for evaluation and improvement of C-CLASS performance. This method accounts of the joint
multiparameter and multiresponse interactions and is suitable for complex land surface schemes, which use large number of parameters. Many of these
conceptual parameters are not directly measurable at the GCM/RCM scales. This approach of model parameter sensitivity analysis provides a way to reduce
the dimensionality of parameter estimation problem. Various observed data sets (listed above) will be used to improve the estimates of C-CLASS
parameters.
F) Optimization of Soil Transfer Scheme for Carbon Studies
As part of the evaluation effort we plan to carry out sensitivity analysis on the number of soil layers used in C-CLASS – i.e. are three soil layers
sufficient? At the present time we can alter the thickness of the soil layers quite easily, but changing the number of soil layers is problematic
given the present formulation for interpolation of soil temperatures through the soil profile. Soil moisture content is computed as an explicit function
of the water balance of each soil layer, but soil temperatures are currently interpolated. As part of the evaluation, we will develop a simple finite
difference scheme for both soil heat and moisture transport to be able to investigate the effect of the number and distribution of soil layers and roots
on the heat, water, and carbon fluxes and stores in C-CLASS. Our analysis will follow the approach used by Martinez et al. (2001).
go to top
Expertise and responsibilities of the terrestrial node members
The effort to develop the terrestrial component of GC3M will be highly integrated. The individual members of the science team will take the lead role
in specific areas of model development, evaluation, sensitivity analysis, and implementation, but all will provide input to each component. Below we
indicate who will take the lead role and who will assist in each aspect of this effort.
Roulet, N. (Theme Leader):
NR expertise is on the role of climate in the cycling of carbon in forested and peatland ecosystems. He has been involved in
the measurement of CO2 and CH4 fluxes from arctic, boreal, and now tropical ecosystems. He and his collaborators have worked extensively with CLASS,
developing the new organic soil parameterization and evaluating its application to wetlands. With collaborators he has developed a carbon model for
peatland ecosystems that has been coupled to CLASS. For the GC3M, in additional to being co-lead PI (see section 11 for his role in project management)
he will lead the terrestrial modelling theme. His scientific responsibilities include developing the parameterization for a northern, poikilhydric PFT;
work on the heterotrophic respiration components (with AA) with particular emphasis how to represent aerobic and anaerobic decomposition pathways in
organic soils and on the production and consumption of methane (with RG); examining the sensitivity of soil climate and carbon turnover to the soil
layering used in CLASS; assist with the development of a scheme to incorporate dynamic vegetation (with DP, AA, RG & AB); and evaluating C-CLASS (with AB)
for wetlands and tropical ecosystems. NR will also supervise the programmer/data manager who will be responsible for the assembly of global data sets,
maintaining the new code in the model, and the archive of all test runs and intercomparison results. To assist in this work he has requested support for
a PDF, a programmer, and a Ph.D. student (see budget). NR will also lead the participation of C-CLASS in EMDI, the ecosystem model intercomparison project.
Arain, A.:
AA expertise is in the flux of energy, heat, water and CO2 between ecosystems and the atmosphere. He has both experience in the measurement and modelling
of the processes that govern these exchanges. He was the senior author of the research version of C-CLASS which first incorporates photosynthesis, and
autotrophic and heterotrophic respiration for application to specific BOREAS sites (Arain et al., submitted). His scientific responsibilities will be to
further develop the photosynthesis and autrotrophic respiration components of C-CLASS particularly for the PFTs used in the global model; to work on the
coupling of autotrophic respiration and heterotrophic respiration (with RG, AB & NR); to incorporate a simple representation relating photosynthesis to
nitrogen availability (with RG); to develop a more realistic function for plant phenology (with AB); and to develop the carbon allocation routine (with
RG). To assist in this work he has requested support for a PDF (see budget).
Black, A.: AB has many years experience in the study of the exchanges of heat, water, and CO2 between terrestrial ecosystems and atmosphere. His
scientific responsibilities will be to take the lead role for off-line evaluation of the various version of C-CLASS to be developed over the next four
years against field data sets. AB will also work on aspects related to the modelling of phenology, soil respiration; the partitioning of autotrophic
respiration; and how to distribute Vcmax through the canopy (with AA). To assist with this work he has requested support for two Ph.D. students (see
budget).
Grant, R.:
RG has developed the ecosystem model ecosys that has significantly advanced our understanding of the coupling of ecosystem processes to climate. His,
with his former PDF Shusen Wang, developed an alternative version of CLASS that contained routines to handle carbon. His scientific responsibilities will
be to assist AA in the synthesis of the two version of C-CLASS; to develop the nitrogen cycle component for C-CLASS; and to work on the development of a
trace gas exchange module with NR. To assist with this work he has requested support for a PDF (see budget).
Price, D.: DP has many years experience in the study of forest dynamics. He is currently working on a CFS initiative to introduce vegetation
dynamics into the IBIS model to examine the impacts of climate change on Canadian forests. His scientific responsibilities will be to develop and implement
the dynamic vegetation component of C-CLASS (with AA, RG, AB & NR) that incorporate disturbance and land- use change. To this end he will take the lead
role in assembling the available data sets of land conversion rates and frequencies of disturbance. To assist with this work he has requested support for
a PDF (see budget)
Verseghy, D.: DV is the author of CLASS. Her scientific responsibilities will be to work with the other investigators to implement the changes into CLASS
once they have been evaluated. She is specifically responsible for the code for CLASS. She will work closely with AA and NR who will maintain the two
development versions of C-CLASS. She will also be responsible for leading the effort on LSP intercomparison through PILPS.
go to top

Science team
Ken Denman (Theme leader), Greg Flato
Background to ocean carbon modelling and objectives (3 and 5 year)
The oceans (exclusive of bottom sediments) contain approximately 50 times more carbon than the atmosphere and almost 20 times more carbon than in
terrestrial vegetation, soils and detritus (Siegenthaler and Sarmiento, 1993). Moreover, Archer et al. (1998) estimate that the ocean will be the
ultimate sink for about 90% of fossil fuel emissions from human activity. These two points underscore the essential role that the oceans have in
controlling the rate at which atmospheric CO2 will increase as well as the eventual level at which it may stabilize. Models of the earth system that
have atmospheric CO2 as a prognostic (i.e. forecasted) variable currently are either box models or models with limited 2-dimensional zonally-averaged
geometry (e.g. Joos et al., 1999). However, recent studies suggest that for certain key carbon cycle variables resulting from box models differ from
those of more comprehensive ocean circulation models (e.g. Archer et al., 2000). Therefore, it is clear that coupled climate models developed to forecast
future levels of atmospheric CO2 will need to include the ocean carbon cycle embedded in full 3-dimensional ocean general circulation models (OGCMs).
However, the recently completed second phase of the IGBP Ocean Carbon Model Intercomparison Project (OCMIP-2) demonstrates that, even in hindcasting the
penetration of chlorofluorocarbons (a passive tracer) into the deep Southern Ocean, the ocean general circulation models from the 13 participating
institutions gave widely divergent results (Najjar et al., 2001). Thus, development of a coupled ocean carbon – general circulation model will require
not only development of a carbon cycle module, but also improvement in the performance of OGCMs.
The recent state of ocean carbon cycle modelling has been reviewed by Doney et al. (submitted), in the context of the Joint Global Ocean Fluxes
Study. At present there is a broad mix of ocean carbon cycle models that include various representations of biogeochemical processes, coupled to physical
circulation and transport models of varying levels of complexity. The first coupled models with some prognostic biological effects that project future
atmospheric concentrations of CO2 have only recently entered the scientific literature (e.g. Sarmiento et al., 1998; Cox et al., 2000). There is also
a controlled comparison (OCMIP) of over a dozen ocean carbon cycle models where the forcing functions and specification of biogeochemical processes are
highly prescribed, such that the range of behaviours exhibited by these models depends largely on the performance of the underlying ocean circulation
models (Sarmiento et al., 2000; Orr et al., 2001). The model outputs have been compared with ocean tracer data sets. The global survey of carbon system
variables obtained during the World Ocean Circulation Experiment (WOCE) hydrographic program is being intercalibrated to provide a high quality global
data set of the (primarily inorganic) ocean carbon system with which to initialize and evaluate model performance (e.g. Lamb et al., 2001). Data sets
from the Joint Global Ocean Fluxes Study (JGOFS) are also becoming widely available to aid in the development and evaluation of models that represent
marine biota and their role in the ocean carbon cycle (e.g. JGOFS Canada, 2000).
Carbon enters the ocean primarily as a CO2 gas transfer across the air-sea boundary from the atmosphere, but also in small amounts in rain, runoff from
land, and released from sediments on the ocean bottom. Gaseous CO2, upon entering the upper ocean, immediately enters the dissolved inorganic pool (DIC),
a dynamic equilibrium between 3 chemical species, dependent primarily on temperature and alkalinity. Only the aqueous CO2, usually about 5% of the DIC
pool, contributes to the partial pressure of CO2 at the sea surface, which regulates exchange of CO2 between the ocean and the atmosphere. DIC enters
the marine planktonic ecosystem via photosynthesis and some is returned via respiration. The rest is partitioned by the ecosystem into organic forms:
(i) non-sinking dissolved and particulate organic forms (DOC) and (ii) sinking organic particles (POC). DOC is transported and mixed both horizontally,
and, more importantly, vertically away from the sea surface. POC sinks out of the surface layer at rates of meters to hundreds of meters per day, some
eventually being deposited and buried in the sediments at the ocean bottom. Both organic forms are continually remineralized (decomposed) back into DIC.
In the absence of oxygen, especially in the sediments, decomposition of POC may lead to generation of CH4 (methane). Other potential marine biotic
feedbacks to climate, including non-carbon mechanisms, have been reviewed by Denman et al. (1996).
The storage time for carbon in the ocean is controlled by the net rate of photosynthetic fixation, the partitioning by the marine ecosystem into DOC and
POC, the rates of remineralization back to DIC, and the time for DIC to be returned to the surface layer by ocean transport and mixing. Overall, the
dynamics of the ocean carbon system can be thought of as a series of transports and transformations. The transports are physical: particles heavier than
the water around them sink; suspended particles, dissolved compounds and gases are advected and mixed by ocean currents, turbulence, internal waves, etc.
The transformations are chemical and biological. Whereas a CO2 molecule in the atmosphere may have an expected lifetime of many decades, a CO2 molecule
entering the ocean at the sea surface immediately enters a dynamical chemical equilibrium between three forms of inorganic carbon, the sum of which is
referred to as DIC (or TCO2 or CO2). The gaseous CO2 may be transformed into bicarbonate or carbonate ions depending on ambient temperature, alkalinity
(or pH), pressure (depth), and the concentrations of several other chemical species. Primary production by phytoplankton transforms CO2 into organic
carbon compounds and bicarbonate ions into calcium carbonate. Plankton release or excrete other forms of particulate (POC) and dissolved (DOC) compounds.
Bacteria further transform these compounds (usually by oxidation) back into inorganic forms. Figure 5 identifies the major transports (solid lines:
sinking – single ended arrow, advection and mixing – double ended arrows) and transformations (dashed arrows), where 'POC' includes living and non-living
particulate organic carbon.
An ocean carbon module requires inclusion of five separate components:
(1) equations governing the transfer of gaseous CO2 across the air-sea interface;
(2) equations governing the chemical partitioning of DIC into its three components, one of which is aqueous CO2;
(3) equations in the sunlit euphotic layer governing the uptake by the ecosystem of CO2 and partitioning into DIC, DOC and POC components, including control
of the uptake of CO2 by limiting nutrients, usually the macronutrient Nitrogen, but also the micronutrient iron;
(4) remineralization (decomposition) of DOC and POC back to DIC (and the related processes for the limiting nutrients) throughout the water column from the
base of the euphotic layer down to the ocean bottom; and finally, (5) embedding all these dissolved and particulate forms of carbon (and limiting nutrients)
in a dynamic ocean general circulation model. The combined ocean carbon module must be sufficiently dynamic to allow the ocean carbon cycle to change as
the climate and ocean dynamics change, with feedbacks via the net air-sea transfer of CO2 affecting the ocean's ability to accept excess CO2 from the
atmosphere.
Theme objectives
The overall objective of the ocean theme is to develop a coupled biogeochemical carbon/OGCM model for inclusion in the CCCma coupled climate model
(CGCM). To achieve this overall objective, several sub-objectives need to be attained:
- complete tests of the CCCma OGCM3 (a modified version of NCOM from the US National Center for Atmospheric Research) at various resolutions, and simulate
the penetration of atmospheric CFCs into the South Atlantic and Southern Oceans, for comparison with observations and with the 13 models involved in the
recently-completed OCMIP-2 intercomparisons;
- complete diagnostic simulations using OGCM3 with the inorganic carbon cycle as specified in OCMIP and compare results with those obtained by OCMIP
participants;
- participate (jointly with Andrew Weaver, UVic) in OCMIP-3, in which the plan is to replace the nutrient nudging model used in OCMIP-2 with a common
prognostic upper-ocean biogeochemistry model that describes the complexity of ecosystem processes in substantially more detail (Najjar et al., 2001);
- complete development of a 7-component biological module (2 size classes of phytoplankton, 2 size classes of zooplankton, dissolved and suspended organic
matter [DOM], sinking particulate organic matter [POM], and dissolved inorganic limiting nutrient [nitrogen], and interface with the inorganic carbon
module using Redfield C:N stoichiometry; Figure 5) – all in a 1-dimensional column model;
- test the sensitivity of the 1-dimensional coupled model to non-Redfield coupling of the C and N cycles, especially for water column 'remineralization'
of DOM and POM;
- participate in preparation and synthesis of global data sets through OCMIP and other international efforts, e.g. through the North Pacific Marine
Sciences Organization (PICES) for the Pacific Ocean. This work will be hopefully leveraged by addition of a scientist specifically for this purpose
through the federal government climate initiative;
- based on evaluation of 3-5 against common global data sets within OCMIP, select an ocean carbon module in NCOM and couple with existing CGCM model at
the end of year 3; and
- develop a second biogeochemical ocean module incorporating at some level biogenic CaCO3 cycling, nitrogen-fixation, and iron-regulation of organic
carbon sequestration, for coupling with the CGCM in Phase II.
By the end of Phase I (year 3) we expect to have attained objectives 1 – 7 (although especially objective 7 should be an iterative and continual process).
In Phase II our ocean carbon model will be implemented into CGCM along with the dynamic terrestrial carbon model ready for the simulations with the fully
coupled CGCM to be undertaken by the end of Phase II.
Outline of the proposed structure of the ocean carbon model
The proposed ocean carbon model will include the inorganic and organic carbon systems, both embedded in a three-dimensional ocean general circulation model.
Whereas the terrestrial carbon system is separate from the atmospheric module except for an interactive boundary layer effecting the exchange of CO2, the
ocean carbon system is intimately linked to the ocean general circulation. Particularly in the context of model simulations to project climate change over
the next century, the ocean carbon system cannot be treated without considering the changing transports of carbon by ocean currents and mixing processes.
Recent studies (e.g. Archer et al., 2000) demonstrate the dependence of carbon cycle model results on the circulation model used, and that is why such a
high priority must be placed on evaluating the performance of the OGCM in which the ocean carbon module will be embedded. To this end we are performing
a series of control runs with OGCM3, the CCCma ocean circulation model, at different vertical and horizontal resolutions to determine the effects of
resolution on advection of scalars.
Inorganic carbon module
A model of the inorganic carbon system used in OCMIP-2 has been widely distributed and can be implemented in a straightforward manner. However, it
requires iteration to reach a solution for the partial pressure pCO2 which controls exchange between the surface ocean and the lower atmosphere – an
inefficient process on vector super-computers. We are currently evaluating the accuracy of regression approximations to the iterative system and of a
compressed algorithm acquired from the University of Washington.
Biological carbon module
The ecosystem module of the ocean carbon system will require the most development. The Cox et al. (2000) model includes a four-compartment model of the
marine ecosystem (Palmer and Totterdell, 2001), similar to that which we have developed in a one-dimensional mixel layer model (Denman and Peña, 1999,
2001) and which we have used in an OGCM of the Pacific to investigate responses to the 1976 climate shift in the N. Pacific (Haigh et al., 2001). In
order to have dynamic partitioning in time and space of the organic carbon into dissolved/suspended and sinking particles, we are developing a
seven-compartment model shown in Figure 5. At present, we have an intermediate model with partitioning of phytoplankton into two size fractions
according to the total concentration, and with microzooplankton and mesozooplankton, the latter being specified as time dependent grazing according to
long term observations (Denman and Peña, 2001).
There is currently great activity in observing and modelling the effects of the trace element iron (Fe) in regulating the uptake of nitrogen (and carbon)
by marine phytoplankton, primarily in regions of the ocean where nitrogen (N) remains above limiting levels, such as the Southern Ocean and the subarctic
North Pacific. We have used a simple technique for including Fe limitation (Denman and Peña, 1999) which could be used with a geographical template
indicating where Fe is likely limiting (e.g. Fung et al., 2000), but in another project we will be developing a process-based model of Fe limitation
which we might expect to incorporate by year 3. The subtropical gyres are now believed to receive a significant amount of their required N through
fixation of atmospheric N by certain marine planktonic cyanobacteria (e.g. Capone et al., 1997). We are collaborating with scientists at Oregon State
University (Y. Spitz and M. Abbott) who are developing a model of this process. In addition, some species of phytoplankton, e.g. coccolithophorids,
remove calcium carbonate (CaCO3) from surface waters for their skeletons, while actually increasing surface pCO2 because they change the alkalinity.
It is not yet clear how to model this 'calcification' process in the context of the model outlined in Figure 5, but we plan to test the idea of using
fixed but different 'rain ratios' (fraction of CCaCO3:Corganic in the sinking particles at the base of the productive sunlit layer, see Denman et al.,
1996) in the two compartments 'Po1' and 'Po2', with the larger ratio associated with the larger 'bloom' species represented by the fraction 'Po2'. We will
incorporate these 3 processes (N-fixation, Fe-limitation, and calcification) into our 1-dimensional models and will evaluate their performance and
necessity prior to including them in the ocean carbon cycle module in Phase II.>
Coupling the inorganic and biological carbon modules
The biological or ecosystem model is currently expressed in terms of the limiting macronutrient, N. We will initially couple it to the carbon (C) cycle
using the Redfield value for the C:N ratio for both biological uptake of nutrients and remineralization of DOC and POC back into inorganic form. However,
our dynamic remineralization scheme will allow for different remineralization rates for C and for N which would result in shallower recycling of N
relative to C, consistent with observations (e.g. Karl et al., 1996; Denman and Peña, 2000). The biological uptake and the remineralization, both
expressed in terms of C, will then become a sink and a source term in the inorganic carbon module. Some investigators include the effect of biological
uptake and remineralization of [NO3]- (and [NO2]-) on alkalinity. We will determine whether including this term makes a significant difference.
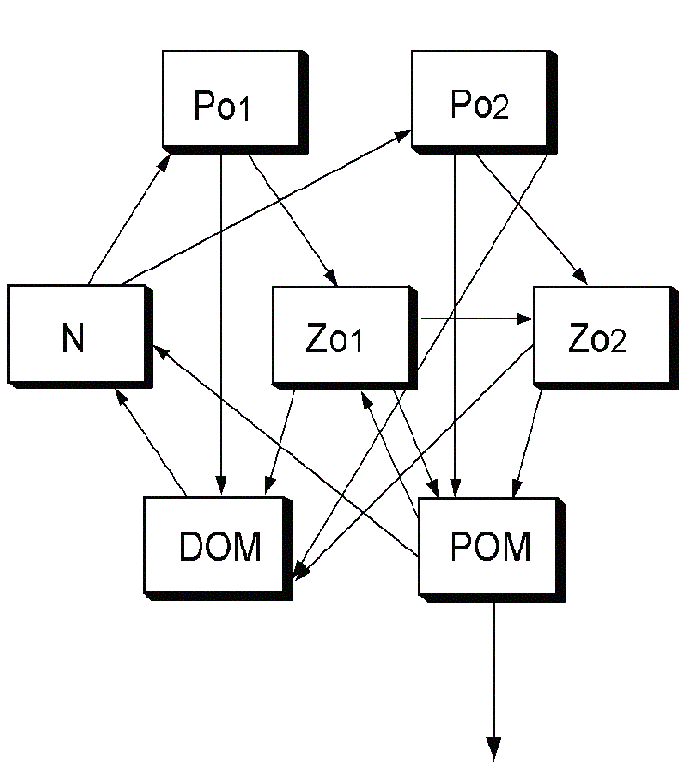
Figure 5. Schematic of the ocean carbon 'biotic pump' module that will be developed and coupled to the inorganic carbon module.
Assembly of global data sets and model evaluation
The CCCma CGCM is compared with other major climate models on a continuing basis through involvement with Working Group I of the Intergovernmental Panel
on Climate Change and other intercomparison activities. In addition, our plans to participate in OCMIP-3 will require comparison of OGCMs in the OCMIP
group (13 groups in OCMIP-2). OCMIP has assembled data sets for evaluating model performance, especially in the transport of passive tracers such as CFCs.
We have worked with the World Ocean Database 98 (see Haigh et al., 2001) and the Comprehensive Ocean Atmosphere Data Set (COADS) and, with our
co-investigators in the atmosphere theme, have a working knowledge of the reanalysis products used to force ocean models.
Through participation in OCMIP-3 we will gain access to the global ocean carbon survey data sets now being compiled (e.g. Lamb et al., 2001). In addition,
as part of the 2000 climate submission to Cabinet, we expect to hire a research scientist to work with the global ocean carbon datasets. Data sets from
JGOFS, needed for development and evaluation of our ecosystem module, are reaching the public domain (KD remains a member of the JGOFS North Pacific
Synthesis Task Team). In addition, KD is a co-PI in a proposal to the US National Ocean Partnership Program called PARADIGM, the PARtnership for Advancing
Interdisciplinary Global Modeling, which has as two objectives: assembling data sets from JGOFS and other programs for developing the next generation of
marine ecosystem models; and intercomparing the series of new models.
Expertise and responsibilities of the ocean theme members
Development of the oceanic component of GC3M will be carried out jointly by the two members, both in the same institution. Because of their complementary
but different areas of expertise, each will take a lead role in different aspects of this effort as described below.
Denman, K.:
KD has many years of experience studying the effects of physical processes on the functioning of the marine planktonic
ecosystem. Over the last decade, he has acquired broad expertise in assessing and studying the role of biogeochemical processes in the ocean carbon
cycle. He was Convening Lead Author in the 1996 IPCC Scientific Assessment of Climate Change of a chapter on biotic responses to environmental change
and feedbacks to climate. He has developed a series of planktonic ecosystem models coupled to 1-dimensional ocean mixed layer models and to a
3-dimensional ocean general circulation model. As part of the CRN Carbon node led by I. Fung he co-supervised two PDFs, one who has implemented the
inorganic carbon system in the CCCma OGCM3, and the other who has investigated scalar (dissolved carbon and nutrients) transport pathways and timescales,
also in OGCM3. In addition to being co-lead PI in GC3M, KD will lead the oceanic modelling theme. His scientific responsibility will be to develop the
biotic pump aspect of the ocean carbon cycle and interface that with the inorganic ocean carbon module. He will work together with GF to integrate those
modules into the ocean general circulation model, to carry out diagnostic testing and analysis of the coupled ocean model, and to work together with the
other theme leaders to couple all the separate modules. He has requested support for a PDF to develop the oceanic carbon module and a part time programmer
(or series of coop students) to implement the modules and assemble test data (see budget).
Flato, G.:
GF is a co-developer of the CCCma series of coupled models and has principal responsibility for its ocean component. He is also
an expert in the modelling of ice dynamics in general circulation models. He will work closely with KD in the integration of the ocean carbon module into
the OGCM, in coupling all the modules in the CGCM coupled model, and in subsequent testing and simulations with the complete model. He will cosupervise
the personnel employed in the ocean carbon theme.
go to top

Science team
G.J. Boer (Theme leader), M. A. McFarlane, and V. Arora.
Background and objectives
Including carbon in the atmospheric component of the coupled model is, at least nominally, relatively straightforward. The intent is to carry CO2 and CH4,
the two most important radiatively active gaseous carbon compounds. As with any prognostic variable in the atmosphere the conservation equation (written
in pressure coordinates for convenience) is:

The LHS represents the explicitly resolved dynamical transport of the quantity while the RHS represents horizontal and vertical subgrid parameterized
fluxes of CO2 together with internal sources and sinks if they exist.
Carbon Dioxide
For carbon dioxide, So is dominated by the anthropogenic source. The weak internal sink Si is due to “rainout” (where CO2 is dissolved in cloud droplets
and precipitated out). There is no appreciable chemical sink for CO2 which has an atmospheric lifetime exceeding 100 years according to Jackobson (1999).
Vertical subgrid fluxes include those due to boundary layer turbulence, turbulent mixing in the free atmosphere, and to convection. The exchange of
atmospheric CO2 with the terrestrial and oceanic components is accomplished most importantly by the flux DV(ps) at the surface and secondarily by rainout.
The AGCM includes general transport equations of the form (1) so what is needed is:
(1) past, present, and projected future anthropogenic sources of CO2,
(2) suitably parameterized subgrid mixing including the effects of convection,
(3) flux matching conditions at the surface, and
(4) a parameterization ofrainout.
Methane
For methane So again includes the anthropogenic source while the internal sink is chemical. The lifetime of in the atmosphere is of the order of a decade
with the largest part of the loss occurring in the stratosphere where it is oxidized through a series of reactions with water vapour as an important
product. As for CO2, the exchange of CH4 with terrestrial and oceanic components is mediated by the vertical subgrid flux.
Methane is well mixed throughout most of the troposphere. However, observations of the distribution of CH4 in the stratosphere show substantial spatial
and seasonal variability (Randel et al., 1998). This is associated with the relatively rapid reduction of its photochemical lifetime with height in the
stratosphere (LeTexier et al., 1988).
The atmospheric requirements for carrying CH4 in the model are similar to those for CO2 with the additional requirement to parameterize the chemical sink.
The main source of water vapour in the stratosphere is the oxidation of CH4 . Throughout much of the stratosphere, two molecules of H2O are produced for
the loss of one molecule of methane (Randel et al., 1998, LeTaxier et al., 1988). Although this relationship between methane loss and water vapour
production is an end product of a series of reactions it is practically useful in that it leads to a simple parameterization of the water vapour source
in the stratosphere (Boville et al., 2001).
We adopt the most straightforward representation Si = XCH4 of a chemical sink proportional to the CH4 concentration where the damping term, , is a
function of latitude, height, and time of the year. Suitable values of will be obtained from the CFCAS funded Global Chemistry and Climate (GCC)
project. The management committee of that project has agreed to facilitate provision of this information.
Other GHGs
N2O is the next most important GHG (other than CFCs) that is affected by human activity. Natural sources are not well known and the anthropogenic
source is thought to be a significant fraction of the natural source. N2O is long lived and well mixed in the atmosphere. Its major sink is
photodissociation in the stratosphere and secondly through chemical reaction there. N2O will be carried in the model in the same fashion as CH4 with its
sink parameterized as a linear loss process in the stratosphere. CFCs have no natural sources and sources are controlled by the Montreal protocol. They
are well mixed in the troposphere. The concentrations of CFCs will be specified based on available projections.
Interaction with radiation
The main purpose of an interactive C-cycle in the CGCM is to incorporate both the forcing and feedback effects of CO2 and CH4. The model’s radiative code
is fully capable of representing the radiative effects of both gases as well as those of N2O and CFCs. At present, GHGs are assumed to be well mixed in
the model. However, the radiative effects of individual gases whose concentrations vary spatially and temporally can be treated without difficulty.
Initialization
It is important to ensure that the simulated atmospheric distributions of these radiatively active gases are realistic before carrying out coupled control
or climate change simulations. An initial atmospheric simulation with specified surface concentrations of CH4, CO2, and N2O will provide atmospheric
distributions of these gases together with the implied fluxes across the surface and, in the cases of CH4 and N2O, the distribution of the parameterized
chemical sinks. These results, which are determined by transport and mixing processes in the model atmosphere and by the parameterized loss processes will
be compared with available observations. The results will also provide information that can be used to calibrate and constrain the fully interactive
representation of surface source/ sink processes.
Transport climate and climate change
Holzer and Boer (2001) study the changes in “transport climate” that accompany a simulated global warming. For a passive tracer, they find that transport
times increase and, as a consequence, near surface tracer concentrations increase near sources. There are implications for pollution and other transport
related questions. Reader et al. (personal communication) investigate the change of the transport climate of dust with potential implications for ocean
fertilization and other aspects of climate change. We propose to repeat and extend these transport studies to investigate the sensitivity transport
climate to the improved parameterizations of mixing and other processes in the new version of the AGCM.
Transport/climate effects will be further studied by repeating the calculations above with specified surface concentrations of CO2, CH4, and N2O.
The result is a differential calculation that provides information on the effect of changes in transport associated with climate change but independent
of the many other feedbacks and interactions that affect GHG concentrations.
Objectives
The overall objectives of the atmospheric component involve model development and testing to allow CO2, CH4, and N2O as active atmospheric components.
This requires information/parameterizations for sources and sinks, flux matching to transfer carbon and nitrogen into and out of the atmosphere, and
studies of transport processes and their changes in the atmosphere. The ultimate aim is a coupled simulation where atmospheric composition is a prognostic
variable and where CO2 and CH4 concentrations affect, and are in turn affected by, climate change.
The theme objectives for year 3 are to:
- obtain/prepare current distributions of sources and sinks of CO2, CH4 and N2O;
- obtain/prepare current distribution of surface concentrations of CO2, CH4, N2O and CFCs;
- develop and test parameterizations for rainout sink of CO2, surface flux matching conditions for CO2, CH4, and N2O, chemical sink of CH4 and N2O;
- perform atmospheric simulations with surface concentrations analysis of distributions and dominant processes, implied surface fluxes,
stratospheric chemical moisture source, and analysis of numerical aspects of tracer transports;
- transport climate integrations; and
- implement the initial version of terrestrial carbon component and test in coupled simulations.
The theme objectives for year 5 are to:
- analyze the transport climate results;
- obtain/prepare the distributions of sources and sinks of CO2, CH4, and N2O and of CFC concentrations based on historical observations and SRES
scenarios;
- couple atmospheric component to terrestrial and ocean components and coupled control integration; and
- begin full coupled climate change integration.
Expertise and responsibilities of the atmosphere theme members
McFarlane, N. A.: NAM has been associated with all phases of the CCCma atmospheric and coupled model development shown in
Figure 2. He is associated with CMAM, the middle atmosphere version of the model, and with CRCM, the regional climate model. He is also currently a
coinvestigator in the GCC project. He will be responsible for model development and especially for parameterizations for which his expertise is particularly
fitting.
Boer, G.J.: GJB has also been associated with all phases of the CCCma models and especially their use for simulating climate
change both globally as well as regionally in association with the RCM. He will be responsible for the transport climate calculations and for analysis
aspects of the atmospheric component.
Arora, V.: VA joined CCCma as a PDF and has now become of term scientist. VA has been involved with the implementation of global
hydrology in the CGCM, and he is therefore quite experienced with CLASS. His primary responsibility will be the implementation of the various global
version of C-CLASS into the CGCM.
Simulations with GC3M
The development and implementation of biological and inorganic cycling of climatically important carbon and nitrogen compounds as active prognostic
components of the CGCM cannot be undertaken in the usual sequential step by step way if timely progress is to be made. Instead, development will proceed
in parallel both in the terrestrial, oceanic, and atmospheric components and in terms of the development, testing, implementation, and assessment of the
carbon and nitrogen budgets in the climate model. The parallel approach demands a series of uncoupled and coupled simulations during development and
testing phase. In particular we divide the simulations into column, uncoupled, and ultimately coupled integrations performed at early, middle, and late
times in the proposal period.
Early simulations (first 2 years):
- column simulations for development/testing of terrestrial components (section 4.1);
- uncoupled atmosphere simulations for development/testing of CO2/CH4/N2O components;
- uncoupled ocean simulations for development/testing of ocean biology/carbon; and
- initial version of terrestrial carbon component tested in coupled terrestrial/atmosphere simulations.
Middle period simulations (years 3, 4):
- uncoupled simulations for development/testing of dynamic vegetation;
- first attempts to couple all components (except dynamic vegetation);
- initialization/spin-up simulations;
- first attempt at control simulation; and
- analysis and assessment.
Late period simulations (year 5):
- first attempts at coupled terrestrial/atmosphere simulations with dynamic vegetation;
- extended control simulation with all components; and
- begin C3GCM climate change simulation.
The initial implementation and testing of the coupled terrestrial/atmosphere (but not ocean because of the long time scales involved) components is
considered to be within reach and will:
- provide initial experience;
li>provide a framework for coupling terrestrial carbon components in the CGCM;
- allow testing of some parameterization choices; and
- provide a basis against which to compare more sophisticated treatments.
This also ensures that the practical, and sometimes difficult, problems and questions that arise in coupling the carbon component with the CGCM do not
appear at the end of the development phase but are treated at an early stage.
There is an initiative referred to as the Coupled Carbon Cycle Model Intercomparison Project (C4MIP). This project is just getting underway. It is our
intention that we would participate in the activities of this intercomparison once we have a fully operational coupled model.
INTERACTIONS AND PARTNERSHIPS
Partners and Users of the Results of the Proposed Network
The proposed network is a partnership between activities at CCCma and the university community. This partnership, and hence the network are necessary
since CCCma does not have the expertise in house to develop a coupled carbon climate model. In particular, the expertise for the terrestrial component
of the model lies in the university community. The main priority of CCCma is to provide the ability to assess climate and the change question.
Dissemination of Results of this Study
The results of this work will be disseminated through refereed scientific journals and presentations a societal meetings (e.g. CMOS, CGU, AGU). The
results of simulations originating from this work will be made available to the climate science community through the posting on the CCCma Website as
is the standard practice. The progress reports (see section 6.2) will be posted on a project Website. We have no public communication plan as such,
but several members of the science team do actively participate in public presentations and meetings, and have written material for the popular press
and newspapers. The results of this work, once implemented as part of the CCCma climate assessment program would be communicated to the public through
the climate science public information program of Environment Canada.
MANAGEMENT AND BUDGETING
management structure
This network contains fewer nodes and few individuals in each node relative to most other research networks. The network brings together three relative
small groups from different disciplinary backgrounds to work together on a project that will produce a communal output, rather than a collection of
individual or group outputs centred around a common theme or question. Therefore, the management requirements for this network are marginal. Nigel Roulet
and Ken Denman will be responsible for the management of the project. NR will be responsible for overseeing the terrestrial carbon node, while KD will
oversee both the ocean and atmospheric nodes based at University of Victoria. They will also be responsible for the assessment of the work of the individual
members relative to the milestones and will take corrective action if necessary. However, the individuals in this project have worked together through
the CRN, and no action of this kind is anticipated. NR has considerable experience with the CLASS model, having been part of the Land Surface Processes
node of the CRN, and he currently leads the Peatland Carbon Study (PCARS). The latter project is coupling CLASS to a peatland ecosystem model (e.g.
Hilbert et al., 2000; Frolking et al., 2001). He also has had considerable project management experience as the senior scientist on the Northern Wetlands
Study, PI of PCARS, and as a CI in BOREAS. KD has published extensively on ocean biogeochemistry and climate change. He has been on the national and
international Scientific Steering Committees for JGOFS, and was a division chief in DFO for five years.
The project office will be located in the Centre for Climate and Global Change Research (C2GCR), at McGill University, Montreal. The office will collect,
post on the Web, and publish printed versions of project progress reports (as part of the C2GCR Research Report series). We will also announce the
publication of the reports and the Website postings through the C2GCR Quarterly – the Centre’s newsletter that has a distribution of over four hundred.
C2GCR will also organize the science team meetings. C2GCR will not require any overhead for this service.
It is anticipated that the funds for this project will come to McGill University and would then be distributed to the institutions of the various
investigators as occurs for a NSERC Research Network or Strategic Grants project. In turn each institution will submit a semi-annual and annual
financial statement to Research Accounting at McGill University. If the process is different for CFCAS grants we will obviously follow the appropriate
guidelines.
Linking Nodes and Science Meetings
Each investigator of each node will be required to submit a one page progress report every six months. These reports will be collected and posted on the
project Website. The project will publish an annual progress report synthesizing the work of the co-investigators. In the progress reports, particular
attention will be paid to progress relative to the milestones. Continued support as part of this project is conditional on submission of the progress
reports.
The network will have two science meetings per year. One science meeting will be specific to each node: terrestrial, ocean and atmosphere. The annual
science meeting will bring together all co-investigators – participation in this meeting is a requirement for continued supported. At least one of NR
or KD will attend all the science meetings.
We have also requested some travel funds to help support the cost of having several of the post-doctoral fellows travel to other co-investigators locations
to promote smoother coupling of the various components of the terrestrial model. They will also travel to CCCma to assist V. Arora with the implementation
of the global version of terrestrial model components in the GCM.
BENEFITS TO CANADA
Canada must contribute to the pool of knowledge about the climate system if we are to benefit from it. This is the case in both the abstract and in the
more concrete sense. For instance, Canada both contributes to and benefits from the collection of climate model results that reflect new scientific
knowledge as well as providing practical guidance to decision makers. Canada’s participation in the IPCC Assessments in a broad array of areas ensures
the necessary connection with the forefront of climate science.
Canada is one of the worlds largest countries in terms of land area and is bounded by three oceans. Canada is a major producer and consumer of fossil
fuels. The natural sources and sinks of Canadian carbon are poorly understood, especially the changes in both the terrestrial and oceanic carbon pools
that will accompany climate. It is hardly necessary to stress the importance of improving our understanding of the global and Canadian carbon budget
and its perturbations under climate change.
The results of the CGCM have been widely used for impact and other studies via the IPCC Data Distribution Centre, the CCCma website, the US National
Assessment and elsewhere. Results from the GC3M will likewise be made widely available and will provide the basis for downscaling, impact, and mitigation
studies of all kinds in support of Canadian climate interests.
INTELLECTUAL PROPERTY
The information resulting from this research will be made available to the public at no charge and with no exercise of priority rights. The
co-investigators will not disseminate the results of work of students under their supervision until the student has had sufficient time to meet their
degree obligations. In addition researchers will refrain from publicizing the results of their work conducted as part of this project until the work
has gone through a peer evaluation. All individuals contributing to the intellectual development of the models in this project will be authors on
relevant papers at a level appropriate to their contribution.
go to top
REFERENCES
Abdella, K., and N.A. McFarlane. 1996. Parameterization of the surface-layer exchange coefficients for atmospheric models. Bound. Lay. Meteor., 80, 223-248.
Aber, J.W.M., et al. 1998. Nitrogen saturation in temperate forest ecosystems: hypotheses revisited. Bioscience 48: 921-934.
Aber, J.E.A. 1989. Nitrogen saturation in northern forest ecosystems. Bioscience 39: 378-386.
Adamsen, A.P.S. and G.M. King. 1993. Methane consumption in temperate and subarctic forest soils: Rates, vertical zonation, and responses to water and nitrogen. Applied Environmental Microbiology 59, 485-490.
Albritton, D.L., and L.G. Meria Fliho. 2001. Techincal Summary of the Working Group I Report. Climate Change 2001: The Scientific Basis. Geneva, World Meterological Organization: 21-83.
Allison, F.E., et al. 1960. The effect of partial pressure of oxygen on denitrification in soil. Soil Sci. Soc. Am Proc. 24, 283-285.
Amthor, J.S. 1984. The role of maintenance respiration in plant growth. Plant, Cell and Environ. 7: 561-569.
Arain M.A., et al. 2000. Effects of Seasonal and Inter-annual Climate Variability on Net Ecosystem Productivity of Boreal Deciduous and Conifer Forests. Canadian J. For. Res. (submitted).
Archer, D., et al. 1998. The dynamics of fossil fuel CO2 neutralization by marine CaCO3. Global Biogeochemical Cycles, 12, 259-276.
Archer, D.E., et al. 2000. Atmospheric pCO2 sensitivity to the biological pump in the ocean. Global Biogeochemical Cycles, 14, 1219-1230.
Arora, V.K. and G.J. Boer. 2001. An assessment of the simulated global moisture budget and the role of moisture reservoirs in processing precipitation. Submitted to J. Geophys. Res.
Arora, V.K. and G.J. Boer. 2001. The effects of simulated climate change on the hydrology of major river basins. J. Geophys. Res., 106, 3335-3348.
Aselmann I. and D.J. Crutzen. 1990. A global inventory of wetland distribution and seasonality, net primary productivity, and estimated methane emissions. In Soils and the Greenhouse Effect. Bouwman A.F. (ed.). pp. 441-449 John Wiley, N.Y.
Barr, A.G., et al. 2000. The phenology of leaf emergence and expansion in a boreal aspen forest. 24th Conf. on Agric. and For Meteorol, Aug 14-18, 2000, Davis, CA, pp 64-65.
Betts, A.K. and J.H. Ball. 1997. Albedo over the boreal forest. J. Geophys. Res., 102, (D24), 28901–28909.
Betts, R.A. 2000. Offset of the potential carbon sink from boreal forestation by decreases in surface albedo. Nature 408(9) 187-190.
Black, T.A., et al.1996. Annual cycles of water vapour and CO2 fluxes in and above a boreal aspen forest. Global Change Biology 2: 219-229.
Black, T.A., et al. 2000. Increased carbon sequestration by a boreal deciduous forest in years with a warm spring. Geophys. Res. Lett. 27: 1271-1274.
Blanken, P.D., et al.1997. Energy balance and canopy conductance of a boreal aspen forest: partitioning overstory and understory components. J. Geophys. Res. 102: 28,915-28,927.
Boer, G.J. 1995. A hybrid moisture variable suitable for spectral GCMS. In Res. Act. Atmos., Ocn. Mod., WMO/TD-No. 665, WMO, Geneva.
Boer, G.J., 2000. Analysis and verification of model climate. Chapter 3 in Numerical Modelling of the Global Atmosphere in the Climate System. Kluwer Academic Publishers, Dordrecht, the Netherlands, 59-82.
Boer, G.J., et al. 2000. A transient climate change simulation with greenhouse gas and aerosol forcing: projected climate for the 21th century. Climate Dynamics, 16, 427-45.
Boer, G.J., et al. 2000. A transient climate change simulation with greenhouse gas and aerosol forcing: experimental design and comparison with the instrumental record for the 20th century. Climate Dynamics, 16, 405-425.
Boer, G.J., et al.1984. The climatology of the Canadian Climate Centre general circulation model as obtained from a five-year simulation. Atmos.- Ocean, 22, 430-473.
Boer, G.J., et al.1984. The Canadian Climate Centre spectral atmospheric general circulation model. Atmos.- Ocean, 22, 397-429.
Bonan, G.B, et al.1992. Effects of boreal forest vegetation on climate. Nature, 359, 716–718.
Bonan, G.B. 1991. Atmospheric-biosphere exchange of carbon dioxide in boreal forests. J. Geophys. Res. 96: 7301-7312.
Bonan, G.B. 1995. Land-atmosphere CO2 exchange simulated by a land surface process model coupled to an atmospheric general circulation model. J. Geophys. Res. 100: 2817-2831.
Bonan, G.B. 1996. A land surface model (LSM version 1.0) for ecological, hydrological and atmospheric studies: Technical description and user guide. NCAR Technical Notes, NCAR/TN-417+STR, National Center for atmospheric Research, Boulder, Colorado.
Bonan, G.B. 1997. Effects of land use on the climate of the United States. Clim. Change, 37, 449–486.
Botta, A., et al. 2000. A global prognostic scheme of leaf onset using satellite data. Global Change Biology 6: 709-725.
Boville, B.A., et al. 2001. Improvements in the NCAR CSM-1 for Transient Climate Simulations. J. Climate, 14, 164-179
Bunnell, F.L., et al.1997. Microbial respiration and substrate weight loss, I, A general model of the influence of abiotic variables. Soil Biol. Biochem. 9: 33-40.
Cao M., et al.1995. Modeling methane emissions from rice paddies. Global Biogeochemical Cycles. 9, 183-195.
Capone, D.G., et al.1997. Trichodesmium, a globally significant marine cyanobacterium, Science, 276, 1221-1229.
Caspersen, J.P., et al. 2000. Contributions of land-use history to carbon accumulation in U.S. forests. Science 290, 1148-1151.
Chen, J-M, et al. 1999. Annual carbon balance of Canada’s forests during 1895-1996. Global Biogeochemical Cycles 14(3) 839-849.
Claussen, M., et al. 2001. Biogeophysical versus biogeochemical feedbacks of large-scale land cover change. Geophysical Research Letters 28(6) 1011-1014.
Collatz, G. J., et al.1992. A coupled photosynthesis-stomatal conductance model for leaves of C4 plants. Australian Journal of Plant Physiology 19: 519-538.
Comer, N. T., et al. 2000. A test of the Canadian Land Surface Scheme (CLASS) for a variety of wetland types. Atmosphere-Oceans 38: 161-179.
Costa, M.H. and J.A. Foley. 1997. The water balance of the Amazon basin: Dependence on vegetation cover and canopy conductance. J Geophysical Research 102, 23 973–23 990.
Costa, M.H. and J.A. Foley. 2000. Combined effects of deforestation and doubled atmospheric CO2 concentrations on the climate of Amazonia. J Climate 13. 18-34.
Cox, P. 2001. Description of the "TRIFFID" dynamic global vegetation model. Brachell, Berks, Hadley Centre, UK Met Office.
Cox, P., et al. 2000. Acceleration of global warming due to carbon-cycle feedbacks in a coupled climate model. Nature 408 184-187.
Cramer, W., et al. 2000. Global response of terrestrial ecosystem structure and function to CO2 and climate change: results of six dynamics vegetation models. Global Change Biology (in press).
Cramer, W., and C.B. Field. 1999. Comparing global models of terrestrial net primary productivity (NPP): introduction. Global Biology Change 19: III-IV.
Cubasch, U., et al., Projections of Future Climate Change. Chapter 9 in the IPCC Third Assessment Report. Cambridge University Press, in press.
Daly, C., et al. 2000. Dynamic simulation of tree-grass interactions for global change studies. Ecological Applications 10(2) 449-469.
Davidson, E. A., et al. 2000. Soil warming and organic carbon content. Nature 408: 789-790.
Denman, K.L., and M.A. Peña, 1999. A coupled 1-D biological / physical model of the northeast subarctic Pacific Ocean with iron limitation, Deep-Sea Research II, 46, 2877-2908.
Denman, K.L., and M.A. Peña, 2000. Beyond JGOFS, pp. 469-490, In: R. Hanson, H. Ducklow, and J. Field (eds), The Changing Ocean Carbon Cycle: A midterm synthesis of the Joint Global Ocean Flux Study, Cambridge University Press.
Denman, K.L., and M.A. Peña, 2001. The response of two coupled 1-D mixed layer / planktonic ecosystem models to climate change in the NE Subarctic Pacific Ocean, Deep-Sea Research II, in revision.
Denman, K.L., et al.1996. Marine biotic responses to environmental change and feedbacks to climate, pp. 483 516, In: Climate Change 1995, J.T. Houghton, L.G. Meira Filho, B.A. Callander, N. Harris, A. Kattenberg and K. Maskell (eds.), Intergovernmental Panel on Climate Change, Cambridge University Press, Cambridge.
Dickinson, R. E., et al.1986. Biosphere-Atmosphere Transfer Scheme (BATS) for the NCAR Community Climate Model. Boulder CO, National Center for Atmospheric Research: 69.
Dickinson, R.E., et al.1998. Interactive canopies for a climate model. J. Clim., 11: 2823- 2836.
Doney, S.C., et al. 2001. Global ocean carbon cycle modeling, In: M.J. Fasham (ed.), JGOFS Synthesis, Cambridge University Press, submitted.
Dunfield, P., et al.1993. Methane production and consumption in temperate and subarctic peat soils: response to temperature and pH. Soil Biology & Biochemistry 25, 321-326.
Ehhalt, D. and M. Prather. 2001. Atmospheric Chemistry and Greenhouse Gases. Climate Change 2001: The Scientific Basis. Cambridge, Cambridge University Press: (in press).
Evans, J.R. 1989. Photosynthesis and nitrogen relationships in leaves of C3 plants. Oecologia 78:9-19.
Faquhar, G.D., et al.1980. A biogeochemical model of photosynthetic CO2 assimilation in leaves of C3 species. Planta, 149: 78-90.
Fechner, E. and H.F. Hemond. 1992. Methane transport and oxidation in the unsaturated zone of a Sphagnum peatland. Global Biogeochemical Cycles. 6, 33-44.
Field, C.B and H.A. Mooney. 1986. The photosynthesis-nitrogen relationship in wild plants. In: On the Economy and Form of Plant Function, (editor) T.J. Givnish, Cambridge University Press, Cambridge, pp. 25-55.
Flannigan, M.D., et al.1998. Future wildfire in circumboreal forests in relation to global warming. J. Vegetation Science 9, 469-476.
Flato, G.M. and G.J. Boer. 2001. Warming asymmetry in climate change simulations. Geophys. Res. Lett., 28, 195-198.
Flato, G.M., et al. 2000. The CCCma global coupled model and its climate. Climate Dynamics, 16, 451-467.
Foley, J.A., et al.1996. An integrated biosphere model of land surface processes, terrestrial carbon balance, and vegetation dynamics. Global Biogeochemical Cycles 10: 603-628.
Friend, A. D., et al.1997. A process-based, terrestrial biopshere model of ecosystem dynamics (Hybrid v3.0). Ecological Modelling 95: 249-287.
Frolking, S. and P. Crill. 1994. Climate controls on temporal variability of methane flux from a poor fen in southeastern New Hampshire: Measurement and modeling. Global Biogeochemical Cycles. 8, 299-327.
Fung, I. Y., et al. 1991. Three-dimensional model synthesis of global methane cycle. Journal of Geophysical Research 96: 13033-13065.
Fung, I., et al. 2000. Full-Form Earth Systems Models: Coupled carbon - climate interaction experiment (the "Flying Leap"). IGBP Newsletter 41: 7-8.
Fung, I.Y., et al. 2000. Iron supply and demand in the upper ocean, Global Biogeochemical Cycles, 14, 281-295.
Gent, P.R. and J.C. McWilliams. 1990. Isopycnal mixing in ocean circulation models. J. Phys. Oceanogr., 20:150-155.
Giardina, C. P., and M.G. Ryan. 2000. Evidence that decomposition rates of organic carbon in mineral soil do not vary with temperature. Nature 404: 858-861.
Goodroad, L.L. and D.R. Keeney. 1984. Nitrous oxide production in aerobic soils under varying pH, temperature and water content. Soil Biology & Biochemistry 16, 39-43.
Gorham, E. 1991. Northern peatlands: Role in the carbon budget and probable responses to global warming. Ecological Applications 1: 182-195.
Gorham, E. 1995. The biogeochemistry of northern peatlands and its possible responses to global warming. Biotic feedbacks in the gobal climate system: Will the warming feed the warming? G. M. Woodwell, and F.T. Mackenzie. New York, Oxford University Press: 169-187.
Gower, S.T., et al. 1997. Carbon distribution and above ground net primary production in aspen, jack pine and black spruce stands in Saskatchewan and Manitoba, Canada. J. Geophys. Res. 102: 29029-29041.
Grant, R.F. 1991. A technique for estimating denitrification rates at different soil temperatures, water contents and nitrate concentrations. Soil Sci. 152:41-52.
Grant, R.F. 1994. Simulation of ecological controls on nitrification. Soil Biol. Biochem. 26: 305-315.
Grant, R.F. 1995. Mathematical modelling of nitrous oxide evolution during nitrification, Soil Biol. Biochem., 27, 1117-1125.
Grant, R.F. 1998a. Simulation of methanogenesis in the mathematical model ecosys. Soil Biol. Biochem. 30:883-896.
Grant, R.F. 1998b. Simulation in ecosys of root growth response to contrasting soil water and nitrogen. Ecological Moelling 107, 237-264.
Grant, R.F. 1999. Simulation of methanotrophy in the mathematical model ecosys. Soil Biol. Biochem. 31: 287-297.
Grant, R.F., et al. 2001a. Long term changes in soil C under different fertilizer, manure and rotation: testing the mathematical model ecosys with data from the Breton Plots. Soil Science Society of America Journal (in press)
Grant, R.F., et al. 2001b. CO2 effects on mass and energy exchange of wheat under different n fertilization: model theory and testing with a Free Air CO2 Enrichment (FACE) experiment. Agron. J. (in press)
Haigh, S.P., et al. 2001. Simulation of the planktonic ecosystem response to pre- and post-1976 forcing in an isopycnic model of the North Pacific, Canadian Journal of Fisheries and Aquatic Sciences, 58, 703-722.
Hänninen, H. 1994. Effect of climatic change on trees from cool and temperate regions: an ecophysiological approach to modelling budburst phenology. Can J. For. Res. 73: 183-199.
Haxeltine, A. and I.C. Prentice. 1996. An equilibrium terrestrial biosphere model based on ecophysiological constraints, resource availability, and competition among plant functional types. Global Biogeochemical Cycles 10(4), 693–709.
Heimann, M., et al. 1998. Evaluation of terrestrial carbon cycle models through simulations of the seasonal cycle of atmospheric CO2: first results of a model intercomparison study. Global Biogeochemical Cycles 12: 1-24.
Hogg, E.H., et al. 2000. Postulated feedbacks of deciduous forest phenology on seasonal climate patterns in the western Canadian interior. J. Climate 13: 4229–4243.
Holland, E.A., et al. 1999. Contemporary and pre-industrial global reactive nitrogen budgets. Biogeochemistry 46: 7-43.
Holzer, M. 1996. Optimal spectral topography and its effect on model climate, J. Clim. 9, 2443-2436.
Holzer, M. and G.J. Boer. 2001. Simulated changes in atmospheric transport climate. Submitted to J. of Climate.
Houghton, R.A., et al. 1983. Changes in the carbon content of the terrestrial biota and the soils between 1860 and 1980: net release of CO2 to the atmosphere. Ecological Monographs 53: 235-262.
Houghton, R.A., et al. 1987. The flux of carbon from terrestrial ecosystems to the atmosphere in 1980 due to changes in land use: geographic distribution of the global flux. Tellus 39B: 122-139.
Houghton, R.A., et al. 2000. Annual fluxes of carbon from deforestation and regrowth in the Brazilian Amazon. Nature 403, 301-304.
Hunter, A.F. and M.J. Lechowicz. 1992. Predicting the timing of budburst in temperate trees. J. Appl Ecol. 29: 597-604.
Indermuehle, A., et al.1999. Holocence carbon-cycle dynamics based on CO2 trapped in ice at Taylor Dome, Antarctica. Nature 398: 121-126.
Itoh, S. and S.A. Barber. 1983. Phosphorus uptake by six plant species as related to root hairs. Agronomy Journal 75, 457-461.
Jackobson, M.Z. 1999. Fundamentals of atmospheric modelling. Cambridge University Press, 656 pp.
Janssens, I. A., 2001a. Productivity overshadows temperature in determining soil and ecosystem respiration across European forests. Global Change Biology 7: 269-278.
Janssens, I.A., et al. 2001b. Forest floor CO2 fluxes estimated by eddy covariance and chamber-based model. Agric. For. Meteorol. 106: 61-69.
JGOFS Canada, 2000. Data Sets 1989-1998. CD-ROM Version 1.0, Marine Environmental Data Service, Canadian Department of Fisheries and Oceans.
Joos, F., et al.1999. Global warming and marine carbon cycle feedbacks on future atmospheric CO2, Science, 284, 464-467.
Karl, D.M., et al. 1996. Seasonal and interannual variability in primary production and particle flux at station ALOHA, Deep-Sea Research II, 43, 539-568.
Kauppi, P. 1996. Carbon budget of temperate zone forests during 1851-2050. In: M.J. Apps and D.T. Price (eds.) Forest Ecosystems, Forest Management and the Global Carbon Cycle. NATO Advanced Science Institutes series, Vol. I40, Springer-Verlag, Heidelberg, pp. 191-198.
King, G.M. and P.S. Adamsen. 1992. Effects of temperature on methane consumption in a forest soil and in pure cultures of the methanotroph Methylomonas rubra. Applied and Environmental Microbiology 58, 2758-2763.
Knorr, W., and M. Heimann. 2001. Uncertainties in global terrestrial biosphere modeling 1. A comprehensive sensitivity analysis with a new photosynthesis and energy balance scheme. Global Biogeochemical Cycles 15: 207-225.
Kucharik, C. J., et al. 2000. Testing the performance of a dynamic global ecosystem model: water balance, carbon balance, and vegetation structure. Global Biogeochemical Cycles 14: 795-825.
Kurz, W.A. and M.J. Apps. 1999. A 70-year retrospective analysis of carbon fluxes in the Canadian forest sector. Ecological Applications 9: 526-547.
Kurz, W.A., et al. 1995. Global climate change: disturbance regimes and biospheric feedbacks of temperate and boreal forests. In: GM Woodwell and FT Mackenzie (eds.), Biotic Feedbacks in the Global Climate System, Oxford University Press, New York, pp. 119-129.
Lamb, M.F., et al. 2001. Consistency and synthesis of Pacific Ocean CO2 survey data. Deep-Sea Res. II, submitted.
Lambert, S.J. and G.J. Boer. 2001. CMIP1 evaluation and intercomparison of coupled climate models. Climate Dynamics, 17, 83-106.
Laprise, R.L., et al.1999. Climate and climate change in Western Canada as simulated by the Canadian Regional Climate Model. Atmos-Ocean, 36, 119-167.
Lavigne, M.B., et al.1997. Comparing nocturnal eddy covariance measurements to estimates of ecosystem respiration made by scaling chamber measurements at six coniferous boreal sites. J. Geophys. Res. 102: 28,977-28,985.
Lessard, R., et al.1994. Methane and carbon dioxide fluxes from poorly drained adjacent cultivated and forest sites. Canadian Journal of Soil Science. 74, 139-146.
LeTexier, H., et al.1988. The role of molecular hyrogen and methane oxidation in the water vapor budget of the stratosphere. Quart. J. Roy. Meteor. Soc., 114,281-295.
Lettau, H., et al.1979. Amazonia’s hydrologic cycle and the role of atmospheric recycling in assessing deforestation effects. Mon. Wea. Rev., 97, 691–699.
Letts, M.G., et al. 2000. Parameterization of peatland hydraulic properties for the Canadian Land Surface Scheme. Atmosphere-Oceans 38: 141-160.
Liang, X., et al. 1998. The Project Intercomparison of Land-Surface Parameterization Schemes (PLIPS) Phase 2c: Red-Arkansas river basin experiment 2. Spatial and temporal analysis of energy fluxes. Global Planetary Change 19: 137-160.
Martikainen, P.J., et al.1993. The effect of changing water table on methane fluxes at two Finnish mire sites. Suo 43, 237-240.
Martinez, J. E., et al. 2001. Effect of the numbers of soil layers on a modeled surface water budget. Water Resources Research 37: 367-377.
McFarlane, N.G., et al.1992. The Canadian Climate Centre second-generation general circulation model and its equilibrium climate, J. Clim. 5, 1013-1044.
McGuire, A. D., et al. 1997. Equilibrium responses of global net primary production and carbon storage to doubled CO2: sensitivity to changes in vegetation nitrogen chemistry. Global Biogeochemical Cycles 11: 173-190.
Meehl, G.A., et al. 2000. The Coupled Model Intercomparison Project (CMIP) Workshop. Bull. Amer. Met. Soc., 81, 313-318.
Melillo, J. M., et al. 1993. “Global climate change and terrestrial net primary production.” Nature 363: 209-240.
Monserud R.A. and R. Leemans. 1992. Comparing global vegetation maps with the Kappa statistic. Ecological Modelling 62, 275-293.
Moore, T.R., and N.T. Roulet. 1995. Methane emissions from Canadian peatlands. Soils and Global Change. R. Lal, J. Kimble, E. Levine, and B.A. Stewart. Boca Raton, Lewis Publishers: 153-164.
Morén, A.-S., and A. Lindroth. 2000. CO2 exchange at the floor of a boreal forest. Agric. For. Meteorol. 101: 1-14.
Murray, M.B., et al. 1989. Date of budburst of fifteen tree species in Britain following climatic warming. J. Appl. Ecol. 26: 693-700.
Nadelhoffer, K.B., et al. 1999. Nitrogen deposition makes a minor contribution to carbon sequestration in temperate forests. Nature 398: 145-148.
Najjar, R., et al. 2001. Predicting the ocean's response to rising CO2: The Ocean Carbon Cycle Model Intercomparison Project, U.S. JGOFS News, 11(1), 1-4.
Nommik, H. 1956. Investigations on denitrification, Acta Agric. Scand., 6, 195-228.
Oechel, W.C., et al. 1994. Transient nature of CO2 fertilization in Arctic tundra. Nature 371: 500-503.
Orr, J.C., et al. 2001. Global Biogeochemical Cycles, 15, 43-60.
Palmer, J.R., and I.J. Totterdell, 2001. Production and export in a global ocean ecosystem model, Deep-Sea Research I, 48, 1169-1198.
Parton, W. J., et al. 1993. Observations and modeling of biomass and soil organic matter dynamics for the grassland biome worldwide. Global Biogeochemical Cycles 7: 785-809.
Parton, W.J., et al. 1987. Analysis of factors controlling soil organic matter levels in Great Plains grasslands. Soil Sci Soc. Am. J. 51:1173-1179.
Pilegaard, K., et al. 2001. Two continuous CO2 eddy-flux measurements over a Danish beech forest Agric. For. Meteorol. 107: 29-41.
Pollard, D., and S.L. Thompson. 1995. “Use of a land-surface-trasnfer scheme (LSX) in a global climate model (GENESIS: The response to doubling stomatal resistance.” Global Planetary Change 7: 129-161.
Poorter, H. and R. Villar. 1997. The fate of acquired carbon in plants: Chemical composition and construction costs. In Plant Resources Allocation, F.A Bazzaz and J. Grace. Eds. Academic Press, 303 p.
Poth, M. and D.D. Focht. 1985. 15N kinetic analysis of N2O production by Nitrosomonas europaea : an examination of nitrifier denitrification. Applied and Environmental Microbiology 49, 1134-1141.
Powlson, D.S., et al. (eds). 1996. Evaluation of soil organic matter models. NATO ASI series. I: Global Environmental Change. Vol. 38.
Prentice, I.C. 2001. The Carbon Cycle and Atmospheric CO2. Climate Change 2001: The Scientific Basis. Cambridge, Cambridge University Press: (in press).
Prentice, I.C., et al. 1992. A global biome model based on plant physiology and dominance, soil properties and climate. Journal of Biogeography 19: 117-134.
Price, D.T. and M.J. Apps. 1996. Summary. In: M.J. Apps and D.T. Price (eds.) Forest Ecosystems, Forest Management and the Global Carbon Cycle. NATO Advanced Science Institutes series, Vol. I40, Springer-Verlag, Heidelberg, pp. 415–421.
Ramankutty, N. and J.A. Foley. 1999a. Characterizing patterns of global land use: North An analysis of globalcroplands data. Global Biogeochemical Cycles 12(4) 667-685.
Ramankutty, N. and J.A. Foley. 1999b. Estimating historical changes in global land cover: North American croplands from 1700 to 1992. Global Biogeochemical Cycles 13(4) 997-1027.
Ramankutty, N., et al. Simulating effects of natural disturbance on forest age structure in a dynamic global vegetation model. (Target journal: Ecological Modelling?).
Randel, W.J., et al. 1998. Seasonal Cycle and QBO Variations in Stratospheric CH4 and H2O Observed in UARS HALOE Data. J. Atmos. Sci., 55, 163-185.
Randerson, J.T., et al. 1996. Substrate limitations for heterotrophs: Implications for models that estimate the seasonal cyclces of atmospheric CO2. Global Biogeochemical Cycles 10: 585-602.
Ruimy, A., et al. 1994. Methodology for estimation of terrestrial net primary production from remote sensed data. Journal of Geophysical Research 99: 5263-5283.
Ryan, M.G., et al. 1997. Annual carbon cost of autotrophic respiration in boreal forest ecosystems in relation to species and climate. J. Geophys. Res. 102: 28871-28883.
Sarmiento, J.L., and S.C. Wofsy. 1999. A U.S. Carbon Cycle Science Plan. Washington DC, U.S. Global Change Research Program: 69 pgs.
Sarmiento, J.L., et al. 2000. Sea-air CO2 fluxes and carbon transport: A comparison of three ocean general circulation models, Global Biogeochemical Cycles, 14, 1267-1281.
Sarmiento, J.L., et al. 1998. Simulated response of the ocean carbon cycle to anthropogenic climate warming, Nature, 393, 245-249.
Schimel, D.S. 1995. Terrestrial ecosystems and the carbon cycle. Global Change Biology 1: 77-91.
Schimel, D.S., et al. 1996. Climate and nitrogen controls on the geography and timescales of terrestrial biogeochemical cycling. Global Biogeochemical Cycles 10: 677-692.
Schimel, D.S., et al. 1997. Continental scale variability in ecosystem processes: models, data and the role of disturbance. Ecological Monographs 67(2) 251-271.
Schlesinger, W.H. 1997. Biogeochemistry: An analysis of global change. San Diego, Academic Press.
Schubert, K.R. (ed.). 1982. The energetics of biological nitrogen fixation. Amer. Soc. Plant Physiol. Rockville, MD. 30 pp.
Sellers, P.J., et al. 1992. Canopy resistance, photosynthesis and transpiration III. A reanalysis using improved leaf models and a new canopy integration scheme. Remote Sensing the Environment 42: 187-216.
Sellers, P.J., et al. 1986. A simple biospheric model (SiB) for use within general circulation models. Journal of Atmospheric Science 43: 505-531.
Shaver, G.R., et al. 1992. Global change and the carbon balance of arctic ecosystems. Bioscience 42: 433-441.
Siegenthaler, U., and J.L. Sarmiento, 1993. Atmospheric carbon dioxide and the ocean, Science, 365, 119-125.
Smid, A.E., and E.G. Beauchamp. Effects of temperature and organic matter on denitrification in soil, Can. J. Soil Sci., 56, 385-391, 1976.
Smith, H.J., et al. 1999. Dual modes of the carbon cycle since the Last Glacial Maximum. Nature 400: 248-250.
Steele, S.J., et al. 1997. Root mass, net primary production and turnover in aspen, jack pine and black spruce forests in Saskatchewan and Manitoba, Canada. Tree Physiol., 17: 577-587.
Stitt, M. 1991. Rising CO2 levels and their potential significance for carbon flow in photosynthetic cells. Plant Cell Environ. 14: 741-762.
Tilman, D., et al. 2001. Forecasting agriculturally driven global environmental change. Science 292: 281-284.
U.S. National Assessment. 2000. Climate change impacts on the United States: Overview. Cambridge University Press, Cambridge, 154 pp.
VEMAP Members. 1995. Vegetation/ecosystem modeling and analysis project: Comparing biogeography and biogeochemistry models in a continental scale study of terrrestrial ecosystem response to climate change and CO2 doubling. Global Biogeochemical Cycles 9: 407-437.
Verseghy, D.L. 1991. CLASS - A Canadian Land Surface Scheme for GCMs. 1. Soil model. International J. Climatology 11: 111-133.
Verseghy, D.L. 2000. The Canadian Land Surface Scheme (CLASS): Its history and future. Atmosphere-Ocean, 38: 1-13.
Verseghy, D.L., et al. 1993. CLASS - A Canadian land surface scheme for GCMs, II. Vegetation model and coupled runs. Int. J. Climatol. 13: 347-370.
Walter, B.P., et al. 2001. Modeling modern methane emissions from natural wetlands 1. Model description. Journal of Geophysical Research (in press).
Walter, B.P., et al. 2001. Modeling modern methane emissions from natural wetlands. 2. Interannual variations 1982-1993. Journal of Geophysical Research (in press).
Walter, B.P., et al. 1996. A process-based model to derive methane emissions from natural wetlands. Hamburg, Germany, Max-Planck-Institut fur Meteorologie.
Wang, Y.P. and Leuning, R. 1998. A two-leaf model for canopy conductance, photosynthesis, and partitioning of available energy. I. Model description. Agric. For. Meteorol. 91: 89-111.
White, A.M., et al. 2000. The high-latitude terrestrial carbon sink: a model analysis. Global Change Biology 6: 227-245.
White, M.A., et al. 1997. A continental phenology model for monitoring vegetation responses to interannual climatic variability. Global Biogeochemical Cycles
Wilson, K.B., et al. 1989. Spatial and seasonal variability of photosynthetic parameters and their relationship to leaf nitrogen in a deciduous forest. Tree Physiol. 20: 565-578.
Zhang, G.J., and N.A. McFarlane. 1995. Sensitivity of climate simulations to the parameterization of cumulus convection in the CCC GCM, Atmos-Ocean, 3, 407-446.
go to top
|
|